James E. Jackson, James F.M. Meaney The non-invasive imaging of blood vessels continues to evolve and there have been significant new developments in cross-sectional imaging techniques since the 5th edition. As discussed in the previous edition of this textbook these have made many diagnostic catheter angiographic techniques almost obsolete; this is a welcome change as they are clearly less invasive and, therefore, safer and will in many instances give more diagnostic information than could be obtained by conventional catheter arteriography because of the concurrent visualisation of surrounding tissues and the ability to reconstruct the data in any plane.1 A good understanding of the basic principles and techniques of catheter angiography remains essential, however, for those intending to become interventional radiologists and this information is still included in this chapter. The newer cross-sectional techniques for imaging blood vessels will, however, be discussed first as these will, quite rightly, be requested before (and often instead of) conventional catheter angiography. Ultrasound plays a key role in a few areas, notably evaluation of the carotid bifurcation and follow-up of peripheral bypass grafts for patency, but it falls to CT and MR to provide detailed information of the vasculature for most other territories. The major advance in CT has been the introduction of multiple rows of detectors, which, in combination with a continuously rotating X-ray source and continuous table movement, allows rapid imaging of a large region of interest during first pass of an intravenously injected bolus. For MR, the key development has been the progressive increase in gradient speed, which, when coupled with parallel imaging that greatly increases acquisition speed, also allows rapid imaging of a large region of interest during first pass of an intravenously injected bolus. MR has the additional advantage that for some indications intravenous contrast injection is not necessary. The development of CT equipment combining a fan-shaped X-ray source, multiple detector rows and continuous table movement has led to the ability to acquire image data from a large tissue volume in a short time period.1 Two major advantages result from rapid CTA acquisition times: firstly, blood vessels of the thorax and abdomen can be imaged during breath-holding; and secondly, it is possible to capture the relatively short ‘arterial’ phase following injection of contrast medium intravenously. Optimal imaging of the vessels during the first arterial passage of contrast material requires both a relatively rapid IV injection of iodinated contrast medium (usually 3–5 mL/s) to ensure adequate arterial opacification, and data acquisition at the appropriate time of vascular enhancement. The latter can be estimated based upon the ‘expected’ time of arrival of the contrast medium within the organ being imaged, but as this varies between patients it can be inaccurate and may result in poor-quality studies. These have been replaced by automated contrast bolus detection techniques in which the ‘arrival’ of contrast medium within a large vessel is measured on images obtained at a single level and data acquisition is initiated when a certain increase in density within the region of interest has been reached. ‘Tight’ boluses of contrast medium using a chaser of normal saline may be useful not only to improve vascular opacification but also to reduce the total volume of contrast medium required. Rapid acquisition of images results in large data sets; for example, comprehensive evaluation of the peripheral vasculature from the level of the renal arteries to the feet with an acquired slice thickness of 0.8 mm will generate approximately 1500 images. Whilst all the diagnostic information is available in this data set, evaluation of the source images is not only extremely time-consuming but also confusing and is helped considerably by reconstruction of the data in axial, coronal and oblique planes without loss of resolution, so-called multiplanar reconstruction (MPR). Tortuous vessels can be ‘straightened’ by curved MPR to aid in the assessment of luminal narrowing caused by, for example, atheromatous disease or encasement by tumour. Maximum intensity projection (MIP) and volume rendering (VR) techniques are additional tools that help greatly in the assessment of blood vessels.2 Each of these reconstruction techniques has its advantages and disadvantages: It should be remembered that, whilst these post-processing techniques are very helpful for diagnostic assessment and for display in multidisciplinary team meetings, the axial source images are essential and allow the operator to distinguish between artefact and disease when an abnormality is suggested on reformatted views. Magnetic resonance angiography is a method for generating images of blood vessels with magnetic resonance imaging (MRI). With improved understanding of the nature of the signals emanating from blood vessels on MR images, it became evident that rather than simply contributing a source of artefacts on MR images, flow phenomena could be harnessed to generate diagnostic ‘angiograms’.3 Although MR angiograms can be generated without use of contrast medium, non-contrast techniques suffer from frequent artefacts and are time inefficient. Contrast-enhanced techniques, which can be completed within seconds rather than the several minutes of earlier methods, have revolutionised clinical practice over the last decade and extended the reach of MRA into all vascular territories with the exception of the coronary arteries.3 TOF angiography relies on the fact that the blood enters the volume under consideration with relatively high velocity and traverses it rapidly, so that it receives very few radio frequency (RF) pulses.3 In order to maximise inflow effect, protons within the imaging volume must be replenished between successive repetition times (TRs), although maximal inflow may not be necessary in clinical practice and some trade-offs can be accepted. Oblique course of the blood vessel being imaged in relation to the slice orientation and short TRs adversely affect signal-to-noise ratios (SNR) as a result of protons under these circumstances experiencing more RF pulses whilst in the imaging slice. The severity and length of stenoses also tend to be overestimated on TOF MRA images because of intravoxel dephasing secondary to turbulent, slow or pulsatile flow.3 As a result of these limitations, TOF MRA has failed to offer a viable non-invasive screening alternative to conventional arteriography, and has not had a major impact on clinical practice outside the brain, where it remains the primary approach for evaluation of the intracranial arteries. Phase-contrast angiography (PCA) is now seldom used in clinical MRA. The methodology underpinning the technique is somewhat complex and, like TOF MRA, images are prone to artefacts and data acquisition is lengthy.4 Because of their unmatched high contrast-to-noise ratios, high spatial resolution, rapid speed of acquisition and relative freedom from artefacts, contrast-enhanced techniques have almost universally replaced non-contrast techniques in clinical practice.5–9 Unlike TOF and PCA techniques, where the intravascular signal is dependent on inherent properties of flow and is, therefore, at the mercy of alterations in flow rate secondary to vascular disease, intravascular signal for contrast-enhanced MRA (CEMRA) depends on a T1 shortening effect induced by the injection of a paramagnetic contrast agent (usually gadolinium based). Images can, therefore, be acquired in any plane, including coronal, which affords the best anatomical coverage for virtually all vascular territories outside the brain.5 In addition, the ability to exploit ultrafast 3D acquisitions (by using the shortest TRs possible and parallel imaging), allows rapid image acquisition that can easily be accommodated within a single breath-hold, an important factor when imaging in the chest and abdomen. In order to generate ‘selective’ arteriograms, images are acquired during the first arterial passage of the contrast agent before its arrival within the veins. The synchronisation of data acquisition with the peak arterial bolus is one of the major challenges of CEMRA, as the rate of transit of contrast medium from the peripheral vein injection site to the vessel of interest is affected by a number of factors, including heart rate, stroke volume and the presence or absence of proximal steno-occlusive lesions. Although the circulation time can be measured using a test bolus, or can be inferred by making some assumptions about the patient’s cardiovascular status, the process is now automated by employing an MR fluoroscopic approach—a technique that demonstrates contrast medium arrival in real time on the display monitor, thus signalling the appropriate time for data acquisition.7,8 The unique nature of k-space (the array of data from which the final image is generated), whereby the central lines determine image contrast and the peripheral lines determine image resolution, can be uniquely exploited to generate CEMRA images with unrivalled signal-to-noise ratios. In situations where breath-holding is not required (e.g. vascular territories which do not move with respiration such as the peripheral arteries and carotid arteries), assuming acquisition of the contrast-defining central lines of k-space is completed during the arterial peak, the continued collection of resolution-defining peripheral lines of k-space during venous enhancement does not result in venous contamination of the images. CEMRA is now the standard of reference for MRA against which all new techniques must be measured. Because of concerns regarding nephrogenic systemic fibrosis,10 there has been renewed interest in non-contrast techniques for body (extracranial) imaging where TOF and PCA techniques are limited.11,12 A new family of ‘balanced’ techniques, where image contrast reflects the ratio of T1/T2, have been developed which portray the vessels as high signal structures. Background tissues are suppressed and either arteries or veins can be highlighted. In many instances the information provided allows contrast injection to be avoided.11,12 Although CT and MR generate broadly similar angiographic images, the former is still favoured in many instances for a number of reasons: MR remains less widely available than CT especially out-of-hours and requires more expertise and operator input; although the time required for acquisition of first pass contrast-enhanced MRA and CTA is similar, the set-up time for MRA is greater; and it is more difficult to monitor unstable patients in the MR environment.1 It is clear, however, that the role of MRA has markedly increased since the last edition of this book, particularly in elective studies of thoracic and abdominal vessels, and it remains important in patients in whom contrast medium is contraindicated. Because of the relatively large size of these vessels, conditions such as aneurysms, stenoses, aberrant anatomy and the relationship between blood vessels and adjacent tumours are equally well demonstrated with both CTA or MRA (Figs. 84-1 and 84-2).13,14 With improvements in spatial resolution, the blood supply to the thoracic cord can be delineated with either technique, information that is useful to the surgeon and helps prevent spinal cord infarction in patients undergoing repair of thoracic aortic aneurysms. CTA (CTPA – CT pulmonary angiography) has been the technique of choice for detection of pulmonary embolism for at least the last decade (Fig. 84-3).15,16 Advantages include high accuracy compared to catheter angiography in a wide range of reported studies, wide availability (including out of hours), short total examination time, ease of patient monitoring during the study and a high degree of clinician confidence in the test. Although several studies have established high accuracy for MRA compared with pulmonary angiography for the evaluation of suspected pulmonary embolism, it is not widely used clinically17,18 (Fig. 84-4). Reasons for this include a reluctance to refer potentially unstable patients to MRI and the availability of CT pulmonary angiography. Improvements in spatial resolution for MRA and additional techniques, such as MR perfusion and ventilation (mirroring the ventilation and perfusion components of nuclear medicine studies albeit at much higher resolution), offer additional functionality to determine the location and distribution of small emboli and may improve the acceptability of MR for PE.17,18 Assessment of the abdomen in virtually all patients with suspected acute vascular emergencies such as abdominal aortic aneurysm rupture and acute mesenteric ischaemia is performed with CTA but the elective assessment of vascular pathology within the abdomen can be performed with either CTA or MRA.5–7,19,20 Abdominal aortic aneurysm morphology can be equally well assessed by CTA and MRA (external dimensions, tortuosity and relationship to visceral arteries) but calcium within the wall is better demonstrated by CTA; for this reason aneurysm assessment before and after endovascular stenting is usually performed with CTA.19 Thrombosis of the spleno-portal system or IVC is usually detected by performing delayed imaging after acquisition of the arterial phase for CTA or MRA (Fig. 84-5). An advantage of MRA is that venographic images can also be acquired using a non-contrast technique. The assessment of native and transplant renal arteries and the staging of hepatopancreaticobiliary neoplasm is well performed with both CTA and MRA, supplemented by additional standard imaging of the parenchyma as indicated.19,20 CEMRA, however, has many advantages for imaging the renal arteries and proximal mesenteric arteries.10 (Fig. 84-6). Numerous studies and meta-analyses attest to the accuracy of MRA in the assessment of significant visceral artery stenotic disease.8 An additional significant benefit of MRA lies in its ability to measure directly the flow rate to each kidney using a two-dimensional (2D) cardiac-triggered, phase-contrast approach, which facilitates both the assessment of end-organ damage and the likelihood of success of transluminal angioplasty.1 As mentioned above, renewed interest in non-contrast techniques has led to the development of reasonably robust techniques which benefit the patient in avoiding the risks of contrast agents in patients with renal impairment or other contraindication to gadolinium contrast agents.11,12
Angiography
Principles, Techniques and Complications
Introduction
Multidetector CT Angiography (Mdcta) Techniques
Magnetic Resonance Angiography (MRA) Techniques
Contrast Mechanisms
Unenhanced Time-of-Flight (TOF) MRA
Phase-Contrast MRA
Contrast-Enhanced MRA (CEMRA)
New Non-Contrast Techniques
Clinical Applications of CTA and MRA
Thorax
Thoracic Aorta and Great Arteries
Pulmonary Arteries
Abdomen
Abdominal Aorta and Abdominal Veins
Renal, Mesenteric and Hepatic Arteries
Carotid and Vertebral Arteries and Intracranial Arteries
Stay updated, free articles. Join our Telegram channel
Full access? Get Clinical Tree
Angiography
Chapter 84
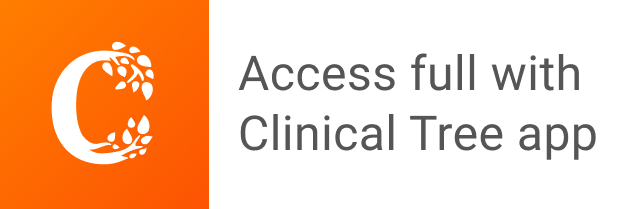