The potential role of cardiac MR imaging in the diagnosis, risk-stratification, and treatment of patients with cardiac arrhythmias is rapidly evolving. Beyond substrate identification and characterization, it is now feasible to perform MR imaging–based noninvasive virtual programmed stimulation to identify potential ablation targets. The next phase of MR imaging–guided electrophysiology may involve intraprocedural guidance of electrophysiologic interventions using real-time MR imaging. This review discusses the spectrum of MR imaging applications in electrophysiology ranging from those that are established in clinical practice to those that are in the experimental phase of development.
Key points
- •
The role of cardiac MR imaging in the diagnosis, risk-stratification, and treatment of patients with cardiac arrhythmias is rapidly evolving.
- •
Beyond substrate identification and characterization, it is now feasible to perform MR imaging–based noninvasive virtual programmed stimulation to identify potential ablation targets.
- •
The next phase of MR imaging–guided electrophysiology may involve intraprocedural guidance of electrophysiologic interventions using real-time MR imaging.
Introduction
Cardiac MR imaging (CMR) has become a cornerstone of the diagnostic and prognostic evaluation of patients with cardiac arrhythmias. However, several limitations currently limit its wider applicability, including artifacts from cardiac implantable electronic devices (CIEDs), safety concerns in patients with CIEDs, and potential toxicities of gadolinium chelates. At the same time, there are many key unmet needs in electrophysiology that CMR is poised to address. These needs include a robust platform for coregistering anatomic and electrophysiologic data in the setting of catheter ablation, a means of distinguishing irreversible injury from edema in the setting of catheter ablation, and a real-time imaging modality that does not involve ionizing radiation to replace intraprocedural fluoroscopy. The aims in this review are, first, to provide an overview of established CMR applications in clinical electrophysiology, and second, to highlight emerging techniques that may be able to address both current limitations of CMR in practice and unmet needs in clinical electrophysiology.
Arrhythmogenic myocardial substrate characterization
CMR offers a variety of tools for identifying and characterizing arrhythmogenic myocardial substrate in both atrial and ventricular arrhythmias. The most established of these is the late gadolinium enhancement (LGE) technique, which provides valuable diagnostic and prognostic information in a variety of substrates. Intravenously administered gadolinium, a heavy metal with differential T1 shortening properties, leads to accumulation in the extracellular space and demonstrates delayed washout from regions with increased interstitial fibrosis. This property facilitates identification, localization, and quantitation particularly of replacement fibrosis using T1-weighted imaging and segmented inversion recovery techniques. These characteristics enable detailed 2- or 3-dimensional characterization of arrhythmogenic substrate in a variety of diseases, including ischemic cardiomyopathy, nonischemic cardiomyopathy (NICM), cardiac sarcoidosis (CS), hypertrophic cardiomyopathy (HCM), arrhythmogenic right ventricular cardiomyopathy, and atrial fibrillation.
In ischemic substrate, the presence, total volume, and transmural extent of LGE provide prognostic information about the risk of subsequent ventricular arrhythmias and death. In NICM, the presence of LGE is a strong prognostic marker of adverse events. A recent meta-analysis of 34 studies that encompassed 4554 patients with nonischemic dilated cardiomyopathy reported a 44.8% prevalence of LGE. Compared with those without LGE, dilated cardiomyopathy patients with LGE had increased odds ratios (OR) for cardiovascular death (OR 3.4, 95% confidence interval [CI], 2.0–5.7), ventricular arrhythmic events (OR 4.5, 95% CI, 3.4–6.0), and recurrent hospitalization for heart failure (OR 2.7, 95% CI 1.7–4.2). The absence of LGE predicted favorable left ventricular (LV) remodeling. A prior meta-analysis reported similar ORs for the predictive values of presence and extent of LGE in both ischemic and nonischemic causes.
In other forms of cardiomyopathy, such as CS, the presence of LGE, especially right ventricular LGE, is associated with an increased risk of adverse events. Coleman and colleagues performed a meta-analysis including 760 patients with CS undergoing LGE-CMR. The presence of LGE conferred an OR of 10.7 for the combined endpoint of ventricular arrhythmia, implantable cardioverter-defibrillator (ICD) shock, sudden cardiac death, or all-cause mortality. Similar relationships have been noted in HCM and acute and chronic myocarditis, which has been reviewed recently.
An emerging technique for improving the prognostic utility of LGE imaging involves using varying signal intensities (SI) within the hyperenhanced area to distinguish regions of core infarct from the so-called border zone or gray zone, and thereby characterize scar heterogeneity. Heterogeneous tissue in the gray zone appears to represent the arrhythmogenic component of postinfarction substrate for ventricular arrhythmias. Specifically, gray zone may harbor critical components of circuitry for reentrant arrhythmias ( Fig. 1 ). Different methods have been described to define core infarct versus gray zone. For instance, core infarct or dense scar may be delineated as a region with SI greater than 3 standard deviations (SD) above that of the null myocardium and gray zone as a region with SI greater than 2 but less than 3 SD above that of the null myocardium. Alternatively, core infarct may be delineated as a region with SI greater than 50% of the peak SI of the total hyperenhanced area, and gray zone is defined as a region with SI greater than peak normal SI or between 35% and 50% of the peak SI. Several studies have shown that gray zone extent was a stronger predictor of subsequent ventricular arrhythmic events compared with core extent, with a recent meta-analysis reporting a pooled relative risk of 5.94 versus 3.82.
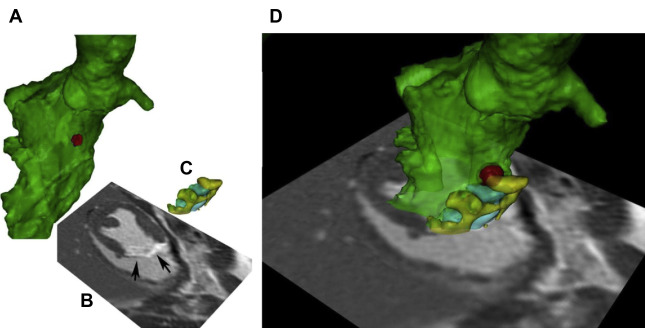
Proof-of-concept studies show that by combining CMR imaging data with assumed information about myofiber orientation, myocyte biology, and tissue conduction properties, a personalized, image-based model of individual patient hearts can be developed ( Fig. 2 ). Such models can be used to perform virtual electrical programmed stimulation as a means of noninvasively identifying inducible substrate for risk stratification as well as for detecting potential targets for catheter ablation in both ventricular and atrial arrhythmias in addition to improving the understanding of mechanisms. Arevalo and colleagues studied patient-specific imaging-based noninvasive programmed stimulation in 41 patients with chronic myocardial infarction and LV ejection fraction ≤35% undergoing primary-prevention ICD placement. Noninvasive inducibility of ventricular arrhythmias conferred a hazard ratio of 4 for appropriate ICD firing or cardiac death, whereas invasive electrophysiology testing conferred a lower hazard ratio of 2.6 for the same combined outcome.
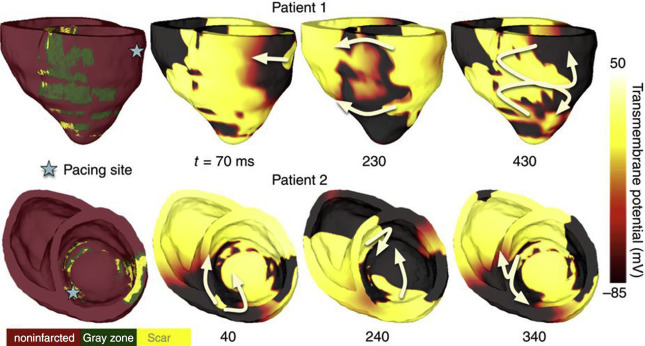
Despite this proliferation of applications, the LGE technique has several important limitations. First, the use of intravenous gadolinium chelates is generally avoided in patients with significant renal dysfunction given concern for systemic nephrogenic fibrosis. Second, reliance on the differential kinetics of gadolinium in different tissues types requires precise timing of image acquisition with respect to contrast administration and precludes rapid serial imaging. Third, because the LGE technique relies on differential SI between areas of fibrosis and normal myocardium, it is best suited to detect replacement fibrosis as opposed to diffuse interstitial myocardial fibrosis. Emerging alternatives to LGE imaging may help to circumvent these challenges.
The native T1 mapping technique is an endogenous contrast method that relies on measurement or estimation of T1 relaxation, which is the course of recovery of longitudinal magnetization. The T1 value depends on intrinsic tissue properties, which differ between normal and diffusely diseased tissue. Methods of estimating T1, rather than obtaining true T1 via turbo spin-echo sequences, enable more rapid image acquisition with fewer breath-holds. The technique appears to be most reproducible when the region of interest is taken from the septum. The technique is less well suited for detecting regional differences in tissue characteristics, but rather for a global assessment of health versus disease in a diffusely altered substrate. The T1ρ technique is another endogenous contrast method that uses a spin-lock sequence to measure T1 relaxation in the rotating frame. This technique appears to be comparable to the LGE technique in differentiating normal remote myocardium from replacement fibrosis.
Cardiac MR imaging–guided electrophysiology intervention
CMR has become an increasingly important tool in the preprocedural, intraprocedural, and postprocedural assessment of patients undergoing catheter ablation for cardiac arrhythmias. Mapping systems that enable merging of imaging data with catheter position and intracardiac electrophysiologic data are now routinely used in clinical practice. Furthermore, the development of experimental MR imaging–compatible catheters that avoid issues with catheter heating, current induction, image distortion, and electromagnetic interference has made intraprocedural MR imaging feasible. Such a platform would enable real-time assessment of anatomy, catheter position, and lesion formation and would limit or eliminate the need for ionizing radiation from fluoroscopy.
Preprocedural Applications
Preprocedural imaging with CMR in anticipation of catheter ablation for cardiac arrhythmias provides helpful information for the electrophysiologist. In routine practice, the LGE-CMR is used to localize areas of probable arrhythmogenesis. LGE-CMR may be especially useful in cases of midwall septal scar, which may not be apparent based on endocardial electroanatomical mapping. Several emerging techniques may provide more precise locations of potential ablation targets.
Conducting channels of viable tissue interdigitating with scar, which form critical slow-conduction components of reentry circuits, may be identified with LGE and may serve as ablation targets. Investigational postprocessing software that identifies these channels has shown good correlation with critical sites identified by invasive electrophysiology study in patients with structural heart disease ( Fig. 3 ). Berruezo and colleagues studied 101 patients with LV scar-mediated ventricular tachycardia (VT). Using preprocedural LGE-CMR to identify potential conducting channels, electrophysiology operators first ablated putative channels and then performed programmed electrical stimulation. If there were residual inducible VTs, the operators proceeded to ablate these. Imaging-based channel identification and dechanneling with radiofrequency (RF) ablation rendered 54.4% of patients noninducible. Further electrogram-guided ablation rendered 78.2% of patients noninducible. Those who required only image-based dechanneling had shorter procedure time, fewer RF ablation lesions, and fewer intraprocedural external cardioversions/defibrillations.
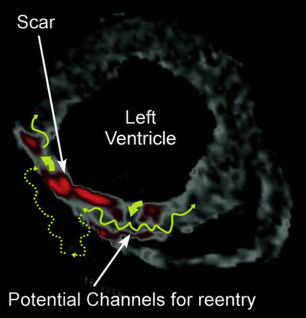
In addition to providing prognostic information, virtual programmed stimulation, described above, may localize targets for catheter ablation in both ventricular and atrial arrhythmias. Ashikaga and colleagues retrospectively studied 11 patients who underwent preprocedural CMR before catheter ablation for VT. They performed image-based simulated programmed stimulation to induce VT. In 9 cases, the simulation-determined ablation target and the invasively determined ablation target regions were the same. In 8 of these cases, catheter ablation was acutely successful, rendering the patient noninducible. An ongoing trial at the authors’ institution, Ablation at Virtual-hEart pRedicted Targets for VT (AVERT-VT; NCT03536052 ), aims to validate this technique in a prospective cohort of patients with ischemic cardiomyopathy who are undergoing catheter ablation for refractory VT. Similar techniques may be applicable in atypical atrial flutter and atrial fibrillation. Zahid and colleagues retrospectively studied 10 patients who underwent preprocedural CMR followed by catheter ablation for left-sided atrial flutter. By use of in silico rapid pacing, left-sided atrial flutter was induced in 7 patients. Simulation-guided ablation targets were identified and compared with actual RF lesions, which were similar in size and location in all 7 patients. In a separate study, Zahid and colleagues retrospectively assessed 20 patients with persistent atrial fibrillation undergoing LGE-CMR. Using 30 virtual pacing sites within the atria, atrial fibrillation was induced in 13 patients and enabled identification and localization of reentrant drivers. There were, on average, 2.7 reentrant drivers per patient. The investigators observed that these drivers were localized in tissue with high fibrosis density and high fibrosis entropy.
Intraprocedural Applications
Catheter ablation traditionally relies on the use of fluoroscopy to determine catheter position. However, fluoroscopy is 2-dimensional, provides limited anatomic detail, and involves administration of ionizing radiation. Limitations with fluoroscopy represent an unmet need in clinical electrophysiology that MR imaging may be poised to address.
At present, the use of intraprocedural MR imaging is limited to static images that are acquired preprocedurally. Preacquired imaging may be used to provide anatomic definition of the cardiac chambers, and associated structures can be used as a “shell” or scaffold with reference to which catheter position can be determined in real time and invasively acquired parameters like voltage can be mapped. These scaffolds can also help operators to avoid potential missteps like ablation within a pulmonary vein. However, this application may be limited by translational shifts in the location of the heart or phasic cardiac motion that creates discordance between the actual locations of structures and their apparent locations on preacquired images.
The development of experimental MR imaging–compatible catheters that circumvent problems with catheter heating, current induction, image distortion, and electromagnetic interference has made intraprocedural MR imaging feasible. These catheters, coupled with emerging noncontrast MR imaging techniques for distinguishing irreversible injury from edema, may permit real-time intraprocedural information about catheter ablation lesions.
Catheter ablation relies on the creation of RF or cryoablation lesions to create controlled, well-defined areas of necrosis. Once ablations are formed, various assays may be performed to define success. For example, following catheter ablation for VT, programmed electrical stimulation may be performed to demonstrate lack of inducible ventricular arrhythmias, which is a common procedural endpoint. Similarly, following pulmonary vein isolation for atrial fibrillation, the absence of pulmonary vein potentials during sinus rhythm or coronary sinus pacing demonstrates pulmonary vein entrance block and is a common procedural endpoint. However, the electrophysiology captured by these assays may reflect the effects of both necrosis and edema from RF or cryoablation lesions. Over time, as edema resolves, the electrophysiologic properties of the lesion may change. Therefore, an intraprocedural means of delineating necrosis versus edema would be useful.
Several groups have shown the utility of combining both T1-weighted and T2-weighted imaging, the latter of which is more specific for edema, to track the time course of ablation lesions and distinguish between necrosis and edema. Although the LGE technique is useful to characterize RF ablation lesions, differential kinetics of gadolinium in different tissues requires careful timing of image acquisition following contrast administration. The ability of noncontrast native T1 mapping approaches to characterize ablation lesions and track their evolution has been reported. A novel, noncontrast T1-weighted technique with long inversion time (TI = 700 ms) permits differentiation of necrosis from edema and permits differentiation of acute from chronic lesions ( Fig. 4 ). With minimal postprocessing, this technique also enables 3-dimensional volume rendering. Finally, because native T1 mapping is a noncontrast technique and does not rely on wash-in and wash-out kinetics of gadolinium chelates, imaging may be performed serially, as needed. A separate technique, proton resonance frequency shift thermography, uses the sensitivity of the proton resonance frequency to temperature and may provide complementary intraprocedural information about RF ablation lesions. Future work in this area could focus on the comparative utility of imaging-based and electrophysiologic assay-based endpoints for ablation procedures in predicting long-term procedural success.
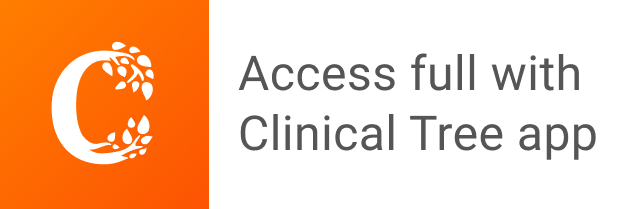