Coronary artery disease (CAD) is a leading cause of global mortality. It results from atherosclerosis, which is a systemic and progressive disease involving the intimal layer of large- and medium-sized arteries. Atherothrombosis, defined as atherosclerotic plaque disruption (predominantly plaque rupture) with superimposed thrombosis, can lead to arterial occlusion and subsequent life-threatening conditions such as acute myocardial infarction (MI) or ischemic stroke. The concept of a “vulnerable plaque” was first introduced to distinguish unstable, rupture-prone plaques from plaques with a stable phenotype. Analyses of human coronary plaque have demonstrated that plaque rupture occurs at a higher frequency than clinical events, suggesting that prothrombotic conditions are also necessary for plaque rupture to trigger acute coronary syndromes. As such, effective risk stratification algorithms need to include both local and systemic factors that confer increased risk of cardiovascular events. At present, clinical risk scoring systems are largely based on the assessment of traditional risk factors with the addition of noninvasive imaging or functional (“stress”) testing for investigation of symptoms that are potentially ischemic in origin. A limitation of this approach includes failure to detect specific features of coronary plaque that confer increased risk of rupture. The identification of high-risk patients might be further improved through the direct assessment of coronary plaque burden, high-risk characteristics, or disease activity, allowing for targeted administration of therapies that are likely to result in prognostic benefits.
Subclinical atherosclerosis can precede the development of clinical disease by many years or even decades, providing an opportunity to identify high-risk patients for targeted primary prevention therapies and ongoing clinical surveillance. At present, multidetector computed tomography (MDCT) coronary angiography is often used for the assessment of low–intermediate risk patients with chest pain where its high negative predictive value allows for the exclusion of coronary artery disease in many individuals. MDCT also allows for the quantification of the overall atherosclerotic plaque burden and the identification of some vulnerable plaque characteristics, through patterns of positive remodeling, low x-ray attenuation, and spotty calcification. Cardiovascular magnetic resonance (CMR) imaging is a noninvasive modality with excellent contrast of soft tissues and the blood/vessel wall interface that has significant potential to assess coronary lumen integrity, plaque burden, and composition without the requirement for ionizing radiation. Advances in CMR data acquisition and gating techniques have improved image quality, which to date has been constrained by limited spatial and temporal resolution. As such, CMR is emerging as a promising modality for coronary artery imaging where its selective use in conjunction with myocardial perfusion imaging, viability, and ventricular function assessments, where CMR is the clinical reference standard, may allow for the complete assessment of suspected CAD with a single imaging modality. Furthermore, evolving molecular imaging techniques may allow for the assessment of disease activity, delineation of vulnerable plaque phenotypes and identification of novel therapeutic targets.
Diagnostic Performance of Coronary Magnetic Resonance Imaging
Studies have shown that coronary magnetic resonance angiography (CMRA) can identify significant coronary artery plaque (>50% stenosis) with a diagnostic accuracy comparable with MDCT. Multiple single center studies have compared the accuracy of CMRA against invasive angiography culminating in a meta-analysis that showed a sensitivity of 87% and specificity of 70% for detection of >50% stenoses. However, technical improvements in coil design, image acquisition/reconstruction, and motion compensation have allowed for a reduction in total imaging time for whole heart CMR coronary angiography to approximately 5 minutes with improved diagnostic accuracy (see Chapter 24 ). Another study has demonstrated that noncontrast, free breathing, three-dimensional (3D) balanced steady-state free precession (bSSFP) whole heart imaging has a sensitivity of 91% and a specificity of 86%, with an area under the receiver operator curve (ROC) of 0.92 when compared with quantitative invasive angiography for the detection of hemodynamically significant plaque. Furthermore, 3D cross-sectional imaging of the coronary vessel wall at 3 T has been able to achieve an in-plane resolution of 0.5 × 0.5 mm, which is comparable with MDCT ( Fig. 28.1 ).
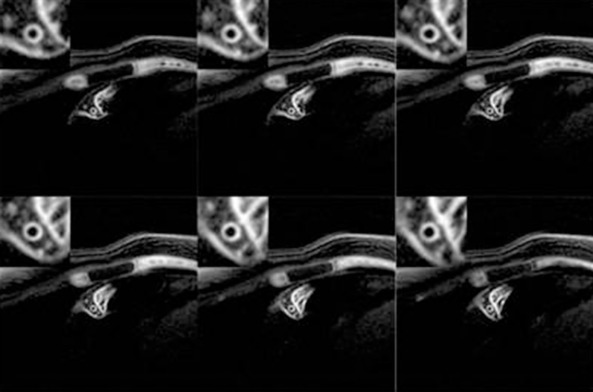
Technical Challenges
Imaging of the coronary vessel wall and lumen is more challenging and technically demanding than imaging of other vascular beds because of the following :
- 1.
Motion: myocardial contraction/relaxation and respiration
- 2.
Small and tortuous vessels
- 3.
Close proximity to epicardial fat, coronary blood, and myocardium
- 4.
Requirement for high spatial resolution
Coronary Magnetic Resonance Imaging Techniques
In both noncontrast and contrast-enhanced CMR, the main limiting factor is imaging time. For coronary artery imaging, a compromise has to be made between imaging time, the spatial resolution achieved, and arterial coverage.
Because a high spatial resolution is paramount for diagnostic coronary artery imaging, longer scan times are necessary, which in turn results in greater susceptibility to motion-related artifacts such as ghosting and blurring. Adequately compensating for motion-induced artifacts is an unsolved problem and one of the technical challenges in CMR that remains an active area of current research.
Motion Compensation Techniques
Cardiac Motion Compensation
Cardiac motion compensation involves synchronizing image acquisition to the electrocardiogram (ECG) to allow for imaging during a motion-free period in the cardiac cycle. The preferred time is the mid diastolic coronary rest period (100–150 ms, approximately 10% of the cardiac cycle), and the exact duration of this interval is heart rate dependent. ECG gating can be performed prospectively (image acquisition limited to mid diastole) or retrospectively, where data are acquired throughout the cardiac cycle but only data from mid diastole are used to generate images ( Fig. 28.2 ). These measures are essential to avoid cardiac-motion induced artifacts but reduce scan efficiency.
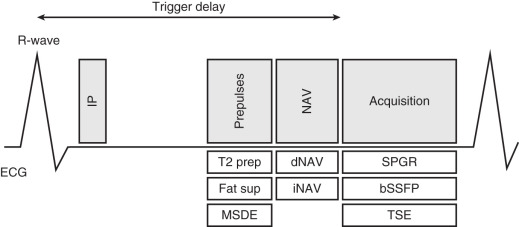
Respiratory Motion Compensation
During normal tidal breathing diaphragmatic excursion results in cardiac motion of up to 20 mm, where the cardiac displacement is many fold greater than coronary vessel thickness (0.5–2 mm). Although breath-holding can be used to suspend respiratory motion for 10 to 20 seconds, this limits the achievable signal-to-noise ratio (SNR) and thus spatial resolution. Three-dimensional coverage of such scans is limited to a thin slab, which reduces the clinical potential as imaging of the entire proximal–mid coronary segments is required to identify prognostically significant disease. Thus coronary artery imaging is usually performed during free breathing with respiratory navigation to compensate for bulk respiratory motion. This is achieved using CMR navigators that monitor the position of the diaphragm with selective data acquisition before image acquisition and restrict image reconstruction usually to data acquired at or near end-expiration (see Chapter 7 ). The respiratory navigators can be unidimensional, two-dimensional (2D), or three-dimensional (3D), where real-time image acquisitions are interleaved with the noncontrast CMR scan and used to compensate for respiratory motion in the CMR acquisition (see Fig. 28.2 ). Unidimensional respiratory compensation typically involves positioning the navigator on the dome of the right hemidiaphragm to measure head–foot motion at the lung–liver interface to perform real-time gating and slice tracking. The use of navigator echoes that record and correct for diaphragmatic motion allows for free breathing and eliminates the time constraints of the breath hold approach, thereby allowing for higher spatial resolution. The respiratory navigators can also be used to track the position of the moving artery by appropriately adjusting the radiofrequency offset and the receiver phase and frequency of the CMR. Because a limited amount of the collected data are used to generate images, image efficiency is reduced and scan time is increased. However, advances in navigator tracking have allowed for larger respiratory gating windows and increased scan efficiency without compromising scan quality. One such technique is the “affine” motion correction, which accurately approximates respiratory-induced coronary motion and, through a 3D geometric transformation, incorporates translation (head–foot movement), rotation, scaling, and shearing. Other limitations of diaphragmatic navigators are the need for a motion model and the need for patient-specific tracking factors. Image-based motion correction with 2D or 3D navigators (iNav; see Fig. 28.2 ) increases motion tracking accuracy because it does not require a motion model as a result of direct measurement of respiration-induced bulk motion of the heart.
Self-Navigation
Self-navigation techniques have been developed to correct for respiratory motion, eliminating the requirement for diaphragmatic tracking. Here, unidimensional self-navigation is used to track the superior–inferior motion of the heart, with segmentation of 3D radial k- space data into different respiratory bins, with respiratory motion correction through a retrospective affine transform enabling 1 mm 3 isotropic resolution and whole heart coverage in 7 minutes. The development of four-dimensional (4D) whole heart, self-navigated sequences for both noncontrast and contrast-enhanced (CE) protocols has allowed for simultaneous assessment of coronary artery anatomy and ventricular function, with the potential for further reductions in scan time. Larger studies are currently awaited to demonstrate the efficacy of these self-navigated and 4D imaging methods.
Accelerated Imaging Techniques
K- Space Filling
K- space filling has a significant impact on image contrast and quality. Cartesian sampling is most commonly used for CMR, but is also the most susceptible to ghosting artifacts. Radial sampling is well suited for motion compensation because the center of k- space (which contains a substantial amount of structural information and image contrast) is repeatedly measured and averaged, with the disadvantage of a lower SNR. Spiral trajectories have been used in studies for both coronary artery lumen and vessel wall CMR but are susceptible to off-resonance artefacts that can lead to image blurring. Non-Cartesian image reconstruction is complex and computationally expensive, especially if used in conjunction with parallel imaging or compressed sensing. Furthermore, repeated measurement of the center of k -space makes radial imaging less sensitive to motion but reduces the impact of prepulses and compromises imaging of tissues with short T1 times (e.g., fat or gadolinium [Gd]).
Pulse Sequences
CMRA requires rapid imaging sequences, such as spoiled gradient recalled echo (GRE) or bSSFP. GRE benefits from administration of an intravenous contrast to generate bright blood angiograms, whereas bSSFP provides high SNR both with and without the use of contrast agents. However, bSSFP is susceptible to magnetic field inhomogeneity-induced black-band artifacts, which has largely restricted its use to field strengths of ≤1.5 T. For imaging at higher field strengths, CE-GRE is favored because it is less susceptible to magnetic field inhomogeneities. GRE in combination with an inversion prepulse is a heavily T1-weighted (T1W) imaging sequence and therefore provides excellent contrast in concert with T1-shortening contrast agents both at 1.5 T and 3 T. T2-weighted and proton-density weighted (PDW) turbo spin-echo sequences have a high SNR and have been used for black-blood vessel wall noncontrast CMR. A limitation of spin-echo techniques is their dependence on blood flow for contrast generation, which can limit their use in patients where coronary blood flow is significantly reduced. Additional preparatory techniques such as T2 prepulses, frequency selective fat suppression and magnetization transfer contrast can also be used to enhance contrast between the vessel lumen and the surrounding fat, myocardium, or venous blood ( Fig. 28.3 ).
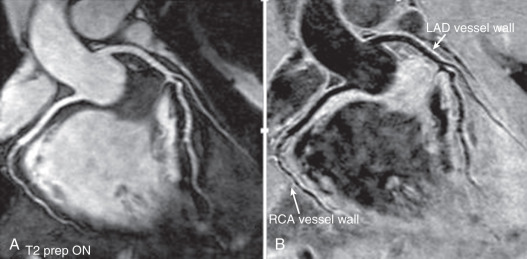
Noncontrast Magnetic Resonance Imaging of Coronary Artery Disease
Noncontrast CMR allows for the direct assessment of:
- 1.
Vascular remodeling
- 2.
Coronary plaque burden
- 3.
High-risk plaque characteristics
Vascular Remodeling and Plaque Burden
Progressive increases in atherosclerotic plaque volume initially lead to positive or Glagovian vascular remodeling, characterized by compensatory enlargement of the outer vessel wall with relative luminal preservation that precludes these changes from being detected by invasive angiography. The total coronary plaque burden and presence of positive vascular remodeling have been shown to be strong predictors of cardiovascular events. Black-blood imaging can be used to quantify plaque burden and this has been demonstrated in patients with subclinical coronary artery disease and type 1 diabetes mellitus in the Multiethnic Study of Atherosclerosis (MESA) cohort ( Fig. 28.4 ). These studies highlight the potential of noncontrast CMR to identify asymptomatic patients with significant plaque burden in the absence of luminal stenosis that would otherwise not be detected with routine functional assessment. At present, cross-sectional 2D black-blood imaging sequences with fat suppression techniques allow for the visualization and quantification of vascular remodeling and plaque burden; however, correlation with intravascular ultrasound (the reference standard) has produced variable results. Challenges of noncontrast coronary vessel wall imaging remain the relatively long scan time, complex planning procedures, and requirement of sufficient coronary flow to generate black-blood images. Recent developments have focused on 3D whole-heart coronary vessel wall imaging with either flow-sensitized or flow independent T2 prepulses.
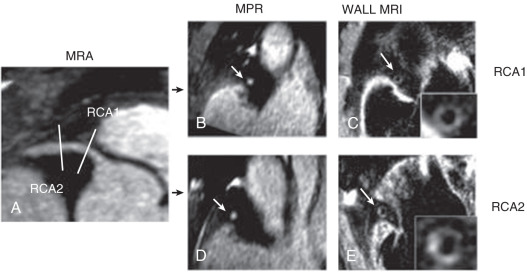
High-intensity plaque (HIP) on T1W imaging was first identified in carotid arteries. The high-intensity signal is considered to represent intraplaque hemorrhage and is associated with recent cerebrovascular events. The signal is postulated to arise from methemoglobin, which is a breakdown product of hemoglobin released 12 to 72 hours following hemorrhage and is a key component of both intraplaque hemorrhage and maturation of thrombus. Methemoglobin contains 5 unpaired electrons, leading to significant shortening of T1 relaxation times of surrounding water molecules. The presence of HIP has been associated with symptomatic cerebrovascular disease, with a positive predictive value of 93% for the identification of complex atherosclerotic lesions with evidence of cap disruption, intraluminal thrombosis, or intraplaque hemorrhage. Translation of T1W imaging of HIP has become feasible for coronary imaging because of advanced motion compensation in combination with T2 preparation, local inversion, or inversion recovery 3D sequences. Because T1W imaging does not yield detailed anatomical images, coregistration with sequences that have superior soft-tissue contrast is required ( Fig. 28.5 ).
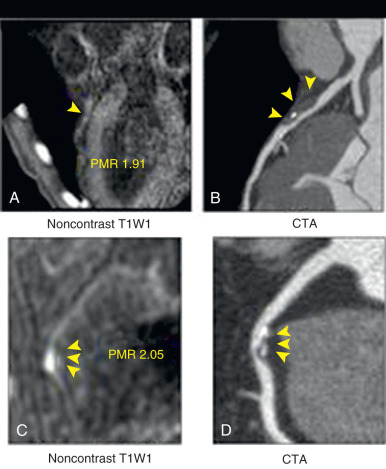
From a coronary viewpoint, the presence of HIP on T1W imaging effectively identifies luminal thrombosis in patients following acute MI with a sensitivity and specificity of >90% ( Fig. 28.6 ). Multimodal imaging has demonstrated that HIP on T1W imaging correlates with positive vascular remodeling, low attenuation, and spotty calcification on coronary CT angiography, which are all features of vulnerable plaque. Furthermore, subsequent prospective studies have demonstrated that HIP with a plaque:myocardial signal ratio (PMR) >1.4 on noncontrast T1W imaging is a significant independent predictor of future coronary events in patients with CAD. The PMR cut off of 1.4 was determined by ROC curve analysis and has a sensitivity of 69.5% and specificity of 82.3% for predicting future cardiac events and statin therapy has been shown to reduce the PMR of HIP. Overall HIP analysis provides prognostic and clinically useful information that is additive to the assessment of overall plaque burden for the improved identification of high-risk patients with CAD.
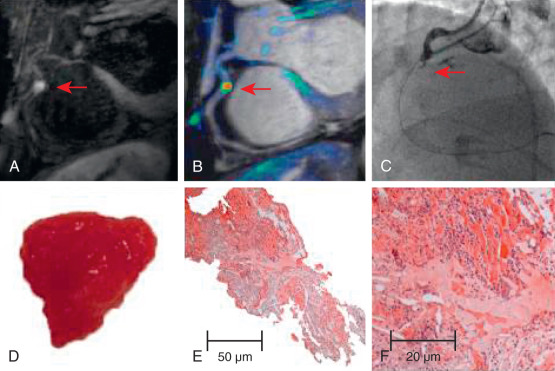
Contrast-Enhanced Magnetic Resonance Imaging of Coronary Artery Disease
CE-CMR using extracellular paramagnetic contrast agents has been studied for the characterization of atherosclerotic plaque. Chelated gadolinium (Gd 3+ ) complexes are the most frequently used signal elements in nontargeted and targeted CMR contrast agents. They act by shortening the T1 time of neighboring free water protons, leading to a detectable signal on T1W (e.g., inversion recovery) sequences, where it produces a positive (bright) signal. These agents are commonly used for imaging the blood pool in contrast-enhanced CMR angiography. Gd contrast agents rapidly redistribute with the surrounding tissue, leaving a 10 to 15 minute window for intravascular (coronary) imaging. They accumulate in both the inflamed vessel wall (increased permeability) and in fibrotic atherosclerotic plaques (increased volume of distribution) ( Fig. 28.7 ). Compared with the previously described double inversion recovery (IR) black-blood coronary vessel wall approaches, nonselective CE-CMR is expected to be less flow sensitive with shorter acquisition times. Assessment of plaque late gadolinium enhancement reduces the imaging task to the presence or absence of contrast uptake in the plaque, with less stringent requirements on spatial resolution than for previously described approaches. The technique also has the potential to show various plaque characteristics such as inflammation and neovascularization. In the context of acute MI, nontargeted Gd results in increased signal in the atherosclerotic vessel wall that diminishes on serial CE-CMR over the ensuing months, possibly indicative of inflammatory regression.
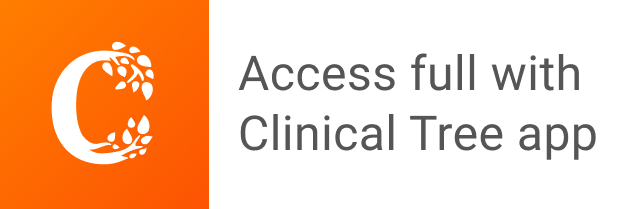