Fig. 1
Schematic of the human auditory cortex. The view is from the top on the superior temporal plane, which is buried inside the Sylvian fissure in the intact brain. The macroscopic anatomy is labeled on the left: Most lateral is the superior temporal gyrus (STG), most of whose surface extends to the lateral surface not seen in this view. Heschl’s gyrus extends from postero-medial to anterior-lateral, where it meets the STG. The border between Heschl’s gyrus and the STG is not sharply defined, and in some brains it appears as if the anterior STG is the continuation of Heschl’s gyrus. Many subjects have more than one Heschl’s gyrus, especially in the lateral part. The Planum temporale starts posterior from Heschl’s gyrus and extends up to the temporo-parietal junction. There is no sharp border between the Planum temporale and the STG.A simplified schematic of histological auditory cortex fields is provided on the right: The core area (also primary auditory cortex, koniocortex, or Brodman Area 41) roughly coincides with the borders of Heschl’s gyrus. Most anatomists subdivide the core region in at least two to three subregions. The nomenclature used here is adopted from the nomenclature used in the monkey (Hackett et al. 2001). The most medial field CM is not always considered a core field. The field A1 is often considered “primary auditory cortex” sensu stricto, but cannot be easily separated from the more lateral field R based on histology. These two fields have opponent tonotopic organizations (Formisano et al. 2003); in A1, high frequencies are localized postero-medially and low frequencies more antero-laterally, and vice versa in R, so that both fields share a common low-frequency border. An alternative nomenclature for the core fields is, from medial to lateral, Te1.1, Te1.0, and Te1.2 (Morosan et al. 2001). The lateral belt is located in the Planum temporale and extends to the lateral surface of the STG (Braak 1978). It can also be subdivided in at least two to three subfields oriented parallel to the core region, but there is only little information available from human anatomy (Rivier and Clarke 1997). Alternative names for areas that overlap with the lateral-belt definition are parakoniocortex, Brodman Area 42, or Te2 (Morosan et al. 2005). The anterior belt field is located in the circular sulcus, anterior from Heschl’s gyrus. This area is also referred to as Prokoniokortex (Galaburda and Sanides 1980). The belt cortex is probably surrounded by the putative parabelt, which includes but may not be limited to Brodman Area 22 or Field Te3 (Morosan et al. 2005); these fields extend far into the lateral STG not seen on the view used here. Note that the different nomenclatures don’t map on each other easily and that there is considerable inter-individual variability
2.2.1 Middle-Latency Auditory Evoked Fields
Most of the spectral energy of the early MAEF lies in the (lower) gamma band around 30–60 Hz, with a maximum around 40 Hz. For recording of the MAEF, the low-pass filter cutoff should therefore not be set below 100 Hz. A high-pass filter with cutoff frequencies in the range of 10–30 Hz is often used to suppress overlapping LAEF components (Fig. 2), because the typical ISI to record the MAEF is around 100–200 ms, and thus shorter than the LAEF. The most prominent peak of the MAEF is the P30m (Pelizzone 1987; Mäkelä et al. 1994; Pantev 1995). The preceding N19m is smaller, but has been consistently localized in Heschl’s gyrus and close to the generator of the P30m (Hashimoto et al. 1995; Gutschalk et al. 1999; Rupp et al. 2002b; Parkkonen et al. 2009). It has been suggested that the N19m and the P30m share the same macroscopic generator in medial Heschl’s gyrus, whereas the P50m is generated more lateral along Heschl’s gyrus (Scherg et al. 1989; Yvert et al. 2001), a view that is supported by depth electrode recordings in patients with epilepsy (Liegeois-Chauvel et al. 1991; Liegeois-Chauvel et al. 1994).
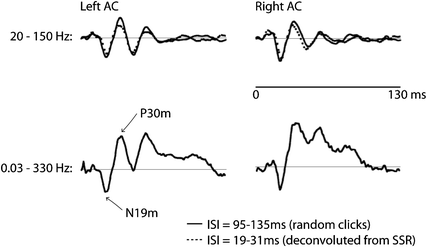
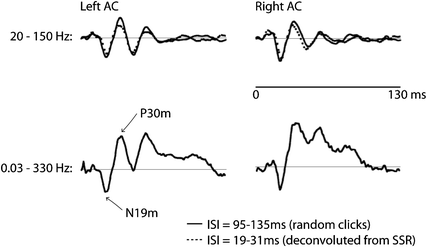
Fig. 2
Middle-latency auditory-evoked fields (MAEF). The data shown are source waveforms based on dipoles in medial Heschl’s gyrus—supposedly in A1—averaged across six listeners. The stimuli were clicks presented with a randomized ISI in the range 95–135 ms (for the data plotted in solid lines). A comparison of two filter settings is shown for the upper (20–150 Hz) and lower (0.03–330 Hz) traces. As can be seen, the peaks N19m and P30m are clearly observed with both setting. The subsequent peaks N41m and P50m are elevated by a slower positivity; the latter is not clearly definable and because of the fast repetition rate might comprise a mixture of slower components. This positive shift has been removed by high-pass filtering in the upper traces. The dotted lines show the waveforms deconvolved from a steady-state response (SSR) with seven different rates (19–31 ms) in the same listeners. Note the high similarity between the early peaks and the traditionally obtained MAEF (Gutschalk et al. 1999)
With reference to microscopic anatomy, the sources of the N19m and P30m are in the auditory core area (Galaburda and Sanides 1980), most likely in the primary auditory cortex field A1. A less-likely alternative is that the N19m and the P30m are generated in the more medial core field CM (Hackett et al. 2001). The more lateral localization of the P50m would better match with a generator in the lateral core field R, but this is more speculative, and it is likely that other fields additionally contribute to the P1m peak measured in MEG (Yvert et al. 2001). Laminar recordings of click-evoked activity in monkey A1 show a peak N8 that is generated in deep cortical layers (4 and 5), and a subsequent P24 which is generated predominantly in layer 3 (Steinschneider et al. 1992). One hypothesis is that human N19m is also generated by thalamocortical input into the granular layer 4.
2.2.2 Long-Latency Auditory Evoked Fields
Traditionally, the earliest peak of the LAEF is the P1m, which has already been mentioned in the context of the MAEF. The earliest latency of the P1m in response to pure-tone stimuli is typically in the range of 50 ms—hence P50m—(Pantev et al. 1996b). There is at least a second subcomponent of the P1 m with a peak latency around 70 ms (Yvert et al. 2001), and for clicks-train stimuli P1m latencies around 60–80 ms are typically observed (Gutschalk et al. 2004a). One reason for P1m variability is that the peak, especially when it is later than 50 ms, overlaps with the onset of the larger N1m, which may reduce the P1m amplitude and latency (Königs and Gutschalk 2012).
By far the most prominent peak of the AEF is the N1m, which comprises a number of subcomponents (Näätänen and Picton 1987) whose specific features are reviewed in more detail below. Optimal recording of the N1m requires an ISI of 500 ms or longer. The spectral content of the N1m lies primarily in the theta band and the lower alpha band (approximately 3–10 Hz), such that low-pass filters down to 20 Hz cutoff frequency can usually be applied without any appreciable effect on component morphology (Fig. 3). High-pass filters are typically chosen in the range of 0.3–3 Hz, depending on the low-frequency noise level and whether later, slower components are also of interest.
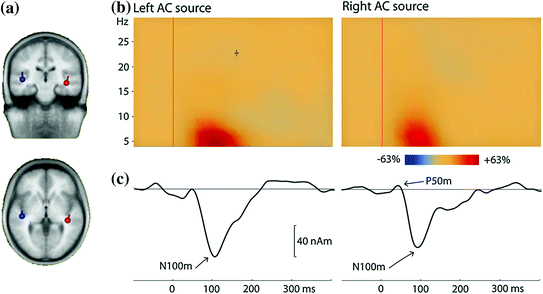
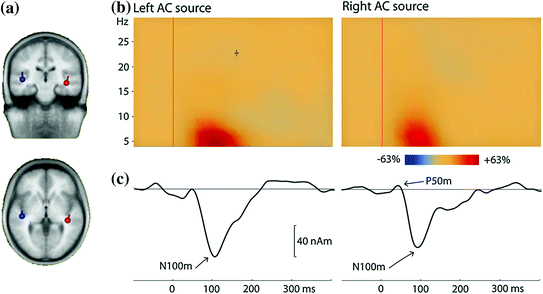
Fig. 3
Long-latency auditory-evoked fields (LAEF) from a single listener. The stimuli were 100-ms long pure tones with frequencies in the range 500–3,000 Hz, presented with a fixed ISI of 800 ms; frequency was randomly changed after 10 s. a Two dipoles were fitted to the averaged data in the time range 80–100 ms. b Time-frequency plots for the time range −100–400 ms and the frequency range 4–30 Hz. The plot shows the enhancement of power in comparison to the baseline in the time interval 100 ms before tone onset. Most of the signal power is in the theta band. c The averaged evoked response is shown for the same time range as the time-frequency analysis (low-pass filter = 30 Hz, no high-pass filter). The most prominent component is the N100m. These source waveforms as well as the time-frequency plots are based on the dipoles shown in (a)
The best-studied subcomponent of the N1m, termed the N100m, peaks at a latency around 100 ms and is generated on the superior temporal plane (Hari et al. 1980). Based on co-registration with anatomical MRI, both Heschl’s gyrus (Eulitz et al. 1995) and the planum temporale, just posterior to Heschl’s gyrus (Lütkenhöner and Steinstrater 1998), are thought to be generators of this subcomponent. One important feature of the N100m is the large ISI range—up to 10 s—below which it will not reach its maximal amplitude (Hari et al. 1982; Pantev et al. 1993; Sams et al. 1993b; McEvoy et al. 1997). This effect is diminished for other N1m subcomponents, which peak at slightly longer latencies (130–150 ms). One subcomponent was localized to the superior temporal gyrus (STG) (Lü et al. 1992), and might be identical to a radial N150 component described in EEG (Scherg and Von Cramon 1986). Another N1m subcomponent has been consistently observed about 1 cm anterior to the main N100m peak and with a latency around 130 ms (Sams et al. 1993b; Loveless et al. 1996; McEvoy et al. 1997; Gutschalk et al. 1998). Note that the latencies of these N1m subcomponent are not fixed but vary considerably with the onset and fine-structure of the stimuli used.
The latency of the subsequent P2m is around 150–250 ms (P200m). Sometimes the P2m has been studied together with the N1m by using a peak-to-peak measure. The few studies that specifically studied the P2m found that the generator of the response is typically located anterior to the N100m (Hari et al. 1987; Pantev et al. 1996a).
For tones longer than about 50 ms, the P2m is followed by a negative wave—the so-called sustained field—whose duration is directly linked to the stimulus duration. To obtain sustained fields, high-pass filters below 0.5 Hz or direct-coupled recordings should be used. The sustained field can be fitted by a dipole source that is typically located anterior to the N100m in auditory cortex (Hari et al. 1980; Hari et al. 1987; Pantev et al. 1994; Pantev et al. 1996a). Based on parametrical variation of sound features such as temporal regularity (see Sect. 3.4) or sound intensity, at least two subcomponents of the sustained field can be separated in lateral Heschl’s gyrus and the planum temporale, similar to the N1m subcomponents (Gutschalk et al. 2002). With respect to microscopic anatomy, the sources of the N1m and the sustained field subcomponents are probably distributed across core and belt fields (Fig. 1).
Importantly, components of the N1m are not only evoked at sound onset from silence, but by all kinds of changes within an ongoing sound (Mäkelä et al. 1988; Sams et al. 1993a). Finally, sounds that are played for a second or longer will also evoke an offset response. This offset response comprises mainly peaks N1m and P2m, whose amplitude varies with sound duration like the onset peaks vary with the silent ISI (Hari et al. 1987; Pantev et al. 1996a).
2.2.3 Selective Adaptation and the Mismatch Negativity
As was briefly noted in the previous paragraph, the N1m amplitude is determined in part by the ISI between the serial tones that are used to evoke the response (Hari et al. 1982; Imada et al. 1997). This observation is based on simple paradigms, where the same sound is repeated once or continuously. The response to each tone is reduced or adapted by the previous tone(s) of the sequence, and more so when the ISI is short. When two different tones are alternated instead, the adaptation of the N1 depends additionally on how different these tones are from each other, as has been shown by several EEG studies (Butler 1968; Näätänen et al. 1988): when pure tones are used, the adaptation is strong when the frequencies of the two tones are near to each other; much less adaptation is observed when the tones are an octave or more apart. This phenomenon is referred to as selective or stimulus–specific adaptation. Selective adaptation is not limited to the N1m, and has more recently been demonstrated for the P1m (Gutschalk et al. 2005).
Another classical auditory stimulus paradigm that uses two tones dissociated by their tone frequency (or other features) is the auditory oddball paradigm (Näätänen et al. 1978). In contrast to the paradigm used to evaluate selective adaptation, the two tones are not simply alternated, but are presented at different probabilities. The more frequent tone is referred to as the standard, whereas the rare tone is referred to as the deviant. The ISI between subsequent tones is typically chosen around 300 ms, where the N1m is almost completely suppressed. In this setting, a prominent negative response with peak latency around 130–200 ms is evoked by the rare deviants, but not by the frequent standard tones. This negative wave, called the mismatch negativity (MMN), is separated from other response components by subtracting the response to standards from the response to deviants. Many studies have examined the MMN and cannot be reviewed here in detail; extensive reviews on this component are already available (Garrido et al. 2009; May and Tiitinen 2010; Näätänen et al. 2011). Briefly, the MMN is not only evoked by differences in tone frequency, but by any sound difference between standard and deviant that is above the listener’s threshold. Originally, the MMN was considered to be a component that is distinct from the other LAEF components reviewed in the previous section. However, this view has recently been challenged: a number of studies suggest that the MMN is identical to the anterior N1m subcomponent, which is reduced in response to the standards but not in response to the deviants due to selective adaptation (May et al. 1999; Jääskeläinen et al. 2004; May and Tiitinen 2010). This view is supported by microelectrode studies in monkey, which suggest that—at least in A1—there is no evidence of an additional evoked component in the context of deviants presented in an oddball paradigm (Fishman and Steinschneider 2012). The associated debate of whether the MMN reflects (bottom-up) selective adaptation (May and Tiitinen 2010), or (top-down) predictive coding (Garrido et al. 2009; Wacongne et al. 2012) is ongoing.
Finally, the MMN itself is not a single component with a stable topography, but comprises at least two subcomponents in the auditory cortex (Imada et al. 1993; Kretzschmar and Gutschalk 2010). Moreover, it has been suggested that the MMN receives contributions from generators in the frontal cortex (Schönwiesner et al. 2007). Second, a subsequent slow negativity that persists for 600 ms is additionally evoked by the oddball paradigm, which is also generated in the more anterior aspect of the auditory cortex along with the generator of the classical MMN (Kretzschmar and Gutschalk 2010).
2.2.4 Auditory Steady-State Responses
The auditory cortex is able to time-lock to periodic stimuli, a phenomenon that has been studied in particular at rates around 40 Hz (Romani et al. 1982; Mäkelä and Hari 1987) (Fig. 4). A periodic brain response that is imposed by a periodic stimulus is referred to as steady- state response (SSR) in EEG and MEG research. Steady-state responses require an evoked component whose inherent spectral power overlaps with the rate of the periodic repetition. As a result, the spectral representation of an SSR is a narrow band at the frequency of the periodic stimulus and sometimes its harmonics. Accordingly, a relationship between the 40-Hz SSR and the early MAEF peaks, whose spectral maximum is close to 40 Hz, was suggested early on (Galambos et al. 1981; Hari et al. 1989), and steady-state responses in the range of 30–50 Hz can be explained by assuming an identical response convolved with the periodic pulse train used as the stimulus. Conversely, when the underlying response is deconvolved on the basis of this assumption (Gutschalk et al. 1999), it shows high similarity with the early MAEF peaks recorded with a transient stimulus paradigm (Fig. 2). The main source of the 40-Hz SSR is in the medial half of Heschl’s gyrus, and thus most likely in the primary area A1 (Fig. 1). This has been demonstrated by source analysis of MEG data (Pantev et al. 1996b; Gutschalk et al. 1999; Brookes et al. 2007), and was confirmed by intracranial recordings (Brugge et al. 2009) and fMRI (Steinmann and Gutschalk 2011). Note that other aspects of the 40-Hz SSR are not readily explained by ongoing, non-refractory MAEF activity. For example, the 40-Hz SSR shows a buildup of activity over about 250 ms before it reaches its constant amplitude (Ross et al. 2002), and this process starts over when, for example, a short sound in another frequency band is presented in parallel (Ross et al. 2005b). Potentially, these effects are related to secondary, more lateral generators of the 40-Hz SSR along Heschl’s gyrus (Gutschalk et al. 1999) up to the superior temporal gyrus (Nourski et al. 2013).
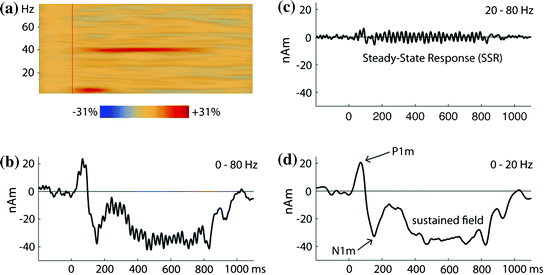
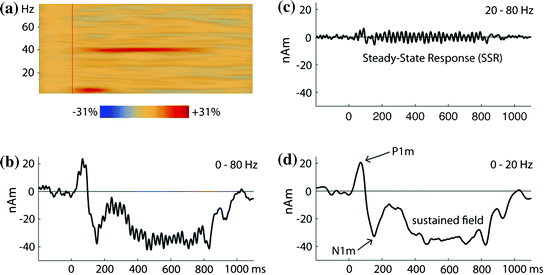
Fig. 4
Auditory 40-Hz steady-state response (SSR) from a single listener. The stimuli were 800-ms long trains of short tone pulses (500 Hz or 1,000 Hz) presented at a rate of 40 Hz. The waveforms are estimated for a dipolar source in the left auditory cortex; highly similar responses were observed on the right (not shown). a Time-frequency plots for the time range −200–1,100 ms and the frequency range 1–80 Hz. The plot shows the enhancement of power in comparison to the baseline in the time interval 200 ms before the train onset. The SSR is seen as narrow activity band at 40 Hz, which persists for the whole stimulus duration. The onset response is reflected by a transient increase of power in the theta band. b Source waveform for the averaged evoked response filtered from 0–80 Hz. In this setting, the LAEF and the SSR are mixed. Because of the broad frequency separation between these components demonstrated in A, they are separated well with different filter settings. c SSR in the frequency range 20–80 Hz, otherwise identical to B. d LAEF in the frequency range 0–20 Hz. Note the strong sustained field that is not captured by the time-frequency analysis
Steady-state responses are not limited to the 40-Hz range: Higher frequency SSRs are observed in relationship to the ABR, known as the frequency following response, but this application has so far been limited to EEG research. In the lower frequency range, it has been demonstrated that SSRs power decreases with increasing modulation rate between 1.5 and 30 Hz (Wang et al. 2012); at the single subject level, a reliable SSR was generally obtained at 1.5, 3.5, and 31.5 Hz, but only variably at 7.5 and 15.5 Hz stimulation rate. The apparently latency was in the range of 100–150 ms, and there was only a weak dependence on the bandwidth of the stimulus carrier. For an SSR at 4 Hz, it was independently demonstrated that the SSR is stronger for stimuli with a non-sinusoidal amplitude modulation and a more rapid sound onset (Prendergast et al. 2010).
2.2.5 Auditory Non-phase-locked Activity
Separating auditory evoked fields from the background activity by response averaging is based on the assumption that there is little or no jitter between subsequent trials. Stronger jitter may blur the shape of the evoked response in the lower frequency (theta) range. In the higher frequency (gamma) range, jitter may easily exceed the phase duration of a single cycle, such that the variable phase relationship between stimulus and response may results in a cancelation of the response by the averaging procedure. Similar response cancelation by averaging occurs for rhythmic activity that appears in a circumscribed time window but not tightly locked to the auditory stimulus. Techniques other than response averaging are required to evaluate such non-phase-looked activity. One possibility is to perform time-frequency analysis on a single-trial level and remove phase information before summation across trials. The increase in response power is typically plotted relative to a pre-stimulus baseline (Figs. 3 and 4). This technique is equally sensitive for phase-locked and non-phase locked activity.
Traditionally, gamma activity in the auditory cortex has been evaluated in a narrow frequency band around 40 Hz (Pantev 1995). More recently, activity in the auditory cortex has been observed in a wide frequency range of 70–250 Hz: this high-gamma activity in human auditory cortex has been clearly demonstrated in intracranial recordings on the superior temporal gyrus (Crone et al. 2001; Edwards et al. 2005; Dykstra et al. 2011) as well as in medial Heschl’s gyrus (Brugge et al. 2009). It has been suggested that high-gamma activity covaries more closely with spiking activity than with evoked potentials in the lower spectral range (Steinschneider et al. 2008; Ray and Maunsell 2011). Measuring gamma activity in the auditory cortex with MEG is more difficult than in the visual system (Kahlbrock et al. 2012; Millman et al. 2013). However, some recent MEG studies raise hope that high-gamma activity can indeed be evaluated non-invasively based on MEG recordings (Todorovic et al. 2011; Sedley et al. 2012).
2.3 Beyond the Auditory Cortex
While activity in the auditory cortex is modulated by active listening, as discussed in more detail in Sect. 4, all response components reviewed so far are readily recorded in a passive mode, where the subject is not attending to the auditory stimulation and may even be involved into reading a book, watching a silent movie, or another task unrelated to the auditory stimulation. Once a task is added that is directly related to the auditory stimulation, however, additional activity can be elicited, the generators of which are supposedly located in multimodal areas beyond the auditory cortex. The most-frequently-studied response elicited during auditory tasks is the P3 or P300. Sources of the P3 have been studied with depth electrodes in patients suffering from epilepsy (Halgren et al. 1998), and in combined EEG-fMRI studies (Linden 2005), suggesting, amongst others, generators in parietal, prefrontal, and cingulate cortex. So far, only a few MEG studies have explored the generators of the P3m, suggesting mostly sources in the temporal and frontal lobes (Rogers et al. 1991; Anurova et al. 2005; Halgren et al. 2011). It remains to be determined, whether P3 generators in other sites are also accessible to MEG. Cortical activity related to auditory cognition beyond the auditory cortex is certainly not limited to the P3, but an extensive review of this topic is beyond the scope of this chapter. The near future will likely bring a wealth of new contributions on the functional relationship between the auditory cortex and areas in the frontal and parietal lobe for auditory cognition.
3 Stimulus Specificity of Auditory MEG Activity
This section reviews a selection of basic sound features and how they are reflected in MEG activity originating in the auditory cortex. Only a brief introduction to the background and psychophysics is provided along with each paragraph, and the reader is referred to the available textbooks on psychological acoustics (Moore 2012), phonetics (Stevens 2000), or auditory physiology (Schnupp et al. 2011) for more details and references to the original publications.
3.1 Temporal Resolution and Integration
Temporal coding of sound is differently reflected in the MAEF and LAEF. The early MAEF peaks are very robust to fast stimulus repetition: When two pulses are repeated at ISIs between 1–14 ms (Rupp et al. 2000), a clear response to the second pulse is observed at ISIs >= 4 ms, and the response is nearly completely recovered at ISIs >= 14 ms. The continuous time-locking capability of the MAEF is also demonstrated by the 40-Hz SSR (Gutschalk et al. 1999; Brugge et al. 2009), which shows phase-locking to inter-click intervals of less than 20 ms.
A classical psychoacoustic paradigm to test temporal resolution is gap detection, where a short interruption in an ongoing sound is used as the stimulus. For example, listeners are able to detect interruptions of few milliseconds duration in a continuous broadband noise. When this stimulus is applied in MEG, gaps as short as 3 ms are sufficient to evoke a significant MAEF response (Rupp et al. 2002a), which is in accordance with psychoacoustic thresholds. Moreover, the higher perceptual thresholds observed at the beginning of a noise burst (5 or 20 ms after onset) are paralleled by a lack of MAEF (Rupp et al. 2004).
The subsequent P1m and N1m are distinctly different with regard to their suppression at short ISI: when periodic click trains are interrupted by omission of one or more clicks, the onset response after the interruption does not show a significant P1m when the interruption is 12 and 24 ms. At gap durations of 48 ms, the P1m is partly recovered, and it has regained almost completely at gaps of 196 ms (Gutschalk et al. 2004b). The time interval required for complete recovery is even longer for the N1m: Some recovery, especially of the anterior N1m generator, is observed between 70–150 ms in a two-tone paradigm (Loveless et al. 1996). With ongoing stimulation, the N1m is reliably observed at ISIs of 300 ms and more (Carver et al. 2002), but some reduction of the response is observed up to 5–10 s (see Sect. 2.2.2). Note that the N1m can also be evoked by all sorts of transients and transitions in ongoing sound, and not only by sound onset. For example, short gaps of 6 ms in an ongoing noise produce not only a P30m, but also a prominent N1m (Rupp et al. 2002a). This should not be mistaken as evidence that the N1m shows similarly fine and fast time-locking as the P30m, but rather reflects the perceptual salience of the transient gap. In contrast to the N19m-P30m, the N1m may also reflect auditory events integrated over longer time intervals. Early studies suggested that the N1m integrates over a time interval of approximately 30–50 ms (Joutsiniemi et al. 1989), because the response amplitude increases with the tone duration for intervals up to this length. More recent studies indicate that temporal integration at the level of the N1m is not captured by a fixed time window and depends on parameters such as the onset dynamics (Biermann and Heil 2000) and temporal structure of the eliciting stimulus (Krumbholz et al. 2003).
3.2 Stimulus Lateralization
Spatial hearing in the horizontal plane is based on two main cues: one is the difference of sound intensity between the ears caused by the head shadow, the interaural level difference (ILD). The other is the timing difference between the ears, or interaural time difference (ITD). For humans, ITD is predominantly used for lower frequencies, whereas ILD is more important for higher frequencies. The relationship between perceived lateralization and the exact physical parameters is variable, depending on the shape and size of the head and ears. To produce spatial hearing perception, arrays of speakers grouped in some distance around the listener in an anechoic room are the gold standard. In MEG, insert earphones are typically used, in which case one relies on direct manipulation of ITD and ILD. Note, however, that this sound delivery produces somewhat non-ecological percepts of sound sources inside of the head. More exact perceptual lateralization with earphones can be achieved with head-related transfer functions, for which the exact physical parameters are measured with microphones placed at the position of the ears. The simplest method of sound lateralization with earphones is monaural presentation, which is again not an ecological stimulus for normal hearing subjects, but can be viewed as an extreme variant of ILD. Moreover, monaural presentation is easy to implement and has a long tradition in experimental psychology and audiology.
Important processing steps of binaural lateralization cues occur early in the brainstem, and are not readily accessible by MEG. Many MEG studies of sound lateralization have instead focused on its effect on the inter-hemispheric balance between the left and right auditory cortex. It was established early on that the N1m evoked by monaural sounds is around 15–30 % larger for contralateral compared to isopilateral stimulation, and that the latency of the N1m is 7–12 ms shorter for contralateral stimulation (Reite et al. 1981; Pantev et al. 1986; Mäkelä et al. 1993). For the P1m, similar amplitude but smaller latency differences in the range of 1–5 ms were reported (Mäkelä et al. 1994). A stronger modulation of response amplitude in the range of 50 % for contra- in comparison to ipsilateral ear stimulation has been observed for the P30m at the sensor level (planar gradiometers), although the effect was smaller in dipole source waveforms (Mäkelä et al. 1994). However, an EEG source analysis study of the N19-P30 found only an amplitude lateralization in the range of 6 % and no latency difference (Scherg and Von Cramon, 1986). Currently, little additional data is available to resolve this discrepancy.
ITDs around the maximal physiological range (700 µs) produce lateralization of N1m-peak amplitudes that can be almost as strong as with monaural presentation (McEvoy et al. 1993; Gutschalk et al. 2012). Moreover, earlier N1m latencies are observed in the auditory cortex contralateral to the perceptual lateralization (McEvoy et al. 1993). In contrast, no significant effect of ITD is observed for the P30m (McEvoy et al. 1994). Recent MEG studies on the coding of ITD in the auditory cortex support a model with a population rate code for opponent left and right channels, in accordance with earlier work in cat (Stecker et al. 2005), by demonstrating that selective adaptation of the N1m depended more strongly on whether the adapter and probe were in the same hemifield than on the actual difference in azimuth (Salminen et al. 2009).
So far, the review of contralateral representation in the auditory cortex is simplified, because the balance of activity between the left and right auditory cortex is not symmetric for left- and right-ear stimulation. An amplitude bias towards the right hemisphere has been observed first for the N1m (Mäkelä et al. 1993), but is even more prominent for the 40-Hz SSR and the sustained field (Ross et al. 2005a): lateralization by ear modulates these responses more strongly in the right AC, and as a result the hemispheric bias is strongly lateralized towards the right auditory cortex for left-ear stimulation and almost counterbalanced for right-ear stimulation (Ross et al. 2005a; Gutschalk et al. 2012). This lateralization bias is not limited to monaural presentation. For example, a combination of ILD and ITD cues, or the use of head-related transfer functions, produces stronger effects on N1m lateralization than either cue alone (Palomaki et al. 2005), but most prominently in the right auditory cortex. Potentially, this right-hemisphere bias is related to a dominant role of the right hemisphere for spatial processing (Kaiser et al. 2000; Spierer et al. 2009). On the other hand, the bias towards the right may be limited to situations where stimuli are presented in quiet, whereas a lateralization bias towards the left has been observed when sounds are presented under perceptual competition (Okamoto et al. 2007a; Elhilali et al. 2009; Königs and Gutschalk 2012
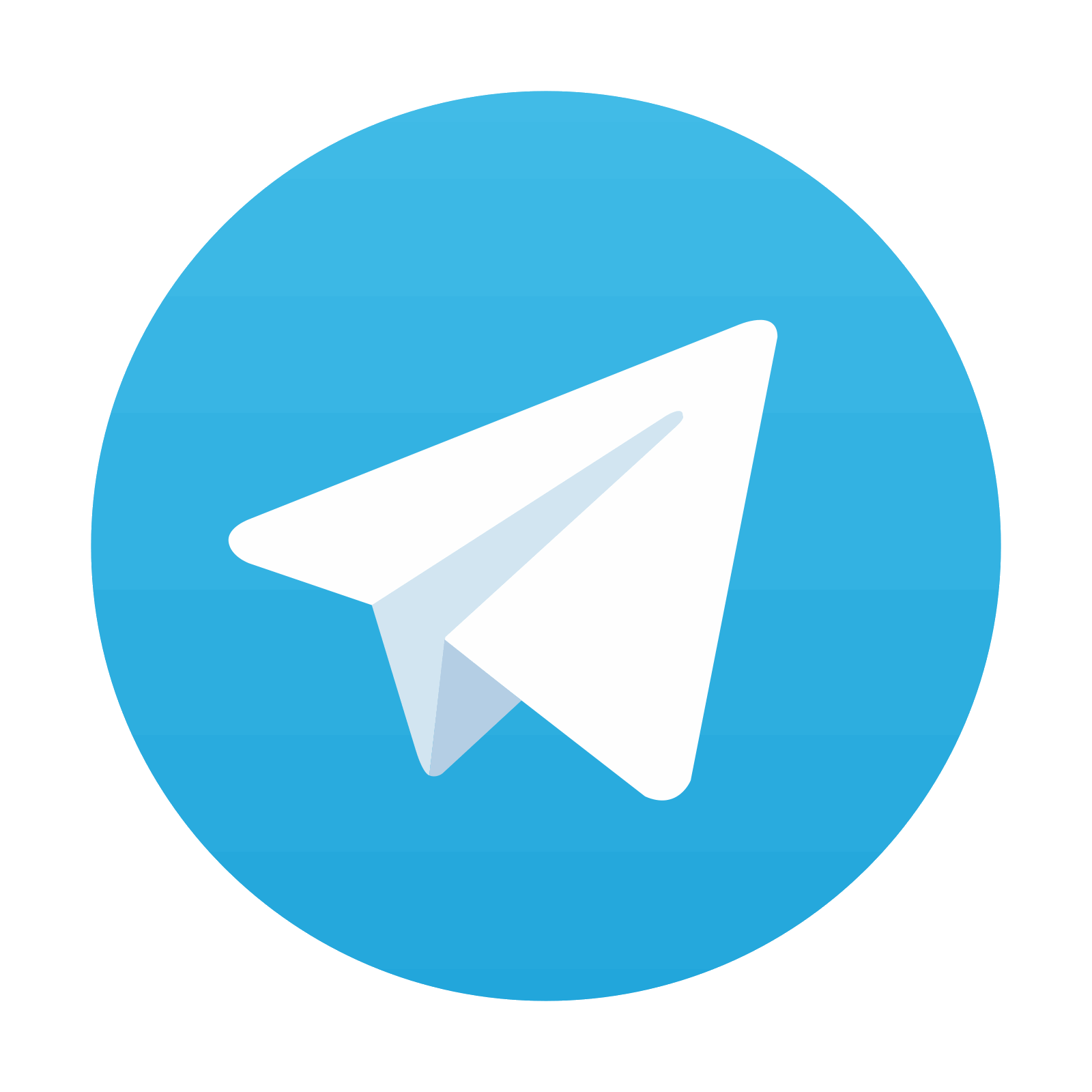
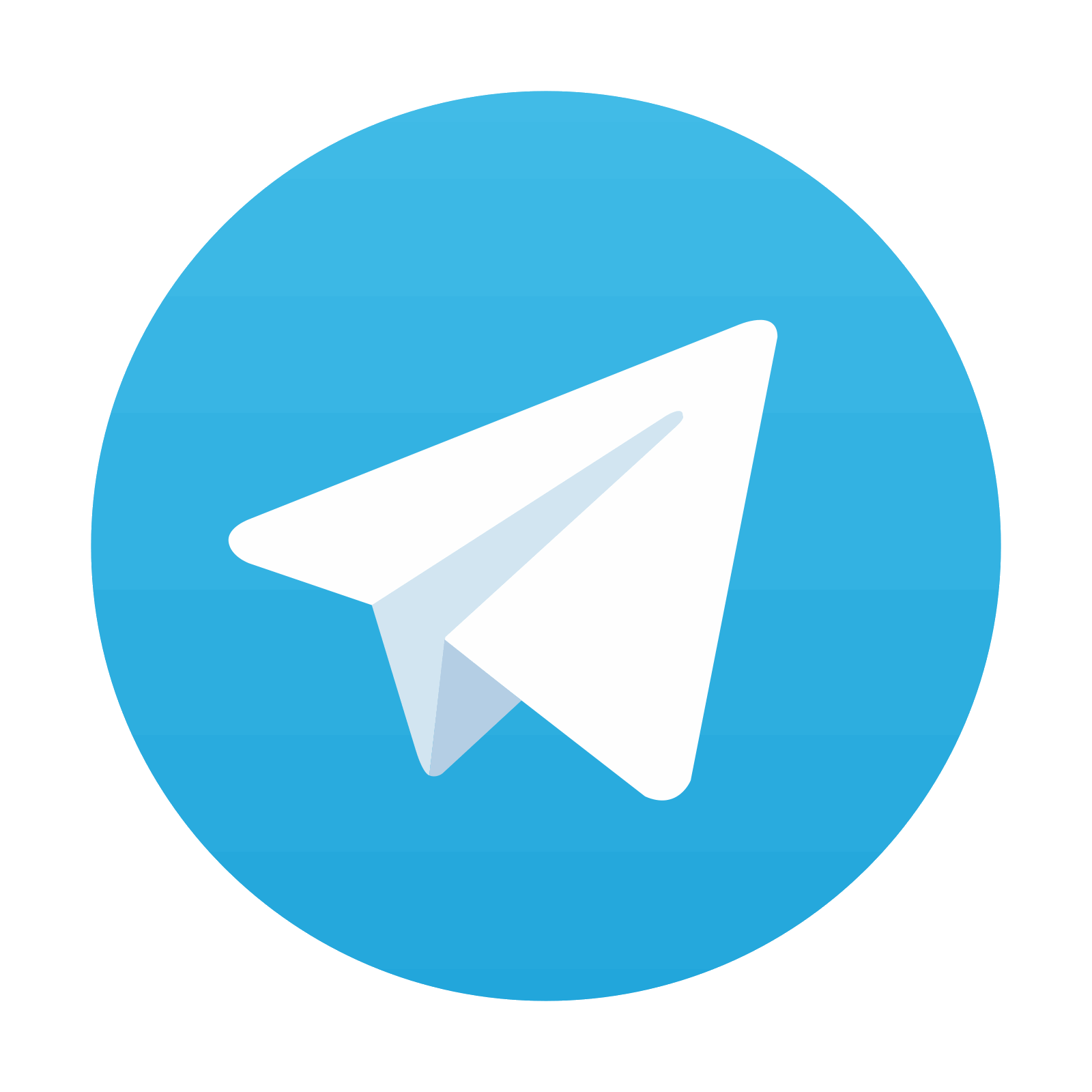
Stay updated, free articles. Join our Telegram channel
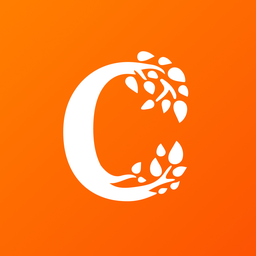
Full access? Get Clinical Tree
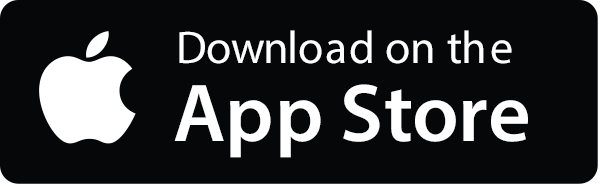
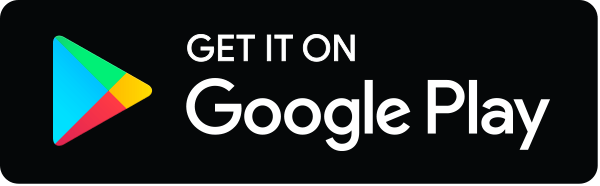