Advances in hardware and software enable high-quality body diffusion-weighted images to be acquired for oncologic assessment. 3.0 T affords improved signal/noise for higher spatial resolution and smaller field-of-view diffusion-weighted imaging (DWI). DWI at 3.0 T can be applied as at 1.5 T to improve tumor detection, disease characterization, and the assessment of treatment response. DWI at 3.0 T can be acquired on a hybrid PET-MR imaging system, to allow functional MR information to be combined with molecular imaging.
Key points
- •
Advances in hardware and software enable high-quality body diffusion-weighted images to be acquired for oncologic assessment.
- •
3.0 T affords improved signal/noise for higher spatial resolution and smaller field-of-view diffusion-weighted imaging (DWI).
- •
DWI at 3.0 T can be applied as at 1.5 T to improve tumor detection, disease characterization, and the assessment of treatment response.
- •
DWI at 3.0 T can be acquired on a hybrid PET-MR imaging system, to allow functional MR information to be combined with molecular imaging.
Introduction
In the past few years, diffusion-weighted imaging (DWI) has become a standard imaging sequence for the evaluation of patients with cancer, especially those on 1.5-Tesla (T) magnetic resonance (MR) systems. This transformation in clinical practice has been brought about by improvements in MR hardware and software, as well as the growing clinical evidence for its use to detect disease, characterize lesions, and assess tumor response to treatment. Furthermore, because the technique is quick to perform, without the need for exogenous contrast administration, it can be routinely deployed for body MR imaging studies without significantly increasing the examination time.
In clinical practice, DWI is largely used as a qualitative tool, although it is a quantitative functional MR imaging technique. Imaging performed at higher diffusion weightings (typically at b-values of 750–1000 s/mm 2 in the body) effectively suppresses the signal from normal background tissue, thus enhancing the conspicuity of cellular disease, which remains high signal intensity. Such qualitative deployment of DWI is widely used in daily practice as a tool to improve tumor detection that may be missed on conventional or contrast-enhanced imaging sequences. However, quantitative DWI is likely to become more important in oncology because these measurements provide objective quantification of water mobility in tissues, which can be applied to aid tissue characterization and to evaluate tumor response to treatment. There is emerging evidence that quantitative DWI can also yield novel predictive or prognostic information.
The apparent diffusion coefficient (ADC), calculated using 2 or more b-values, remains the most widely used quantitative DWI parameter and has been shown to have good measurement repeatability within and between scanners, particularly at 1.5 T. However, there is widening recognition of the nonmonoexponential signal attenuation at increasing b-values in tissues. Consequently, there is now significant interest in applying nonmonoexponential diffusion models to derive additional quantitative parameters that inform on tissue water diffusivity. These nonmonoexponential diffusion models include intravoxel incoherent motion (IVIM), stretched exponential, and diffusion kurtosis models. To enable these models to be used with confidence, there is a need for meticulous image acquisition using more than 2 b-values, to ensure that images have good signal/noise ratio and to ascertain their measurement repeatability. For example, it has been shown that the perfusion-sensitive parameters of perfusion fraction (f) and pseudodiffusion coefficient (D*) have poor measurement repeatability, particularly in hypovascular lesions, which has limited wider translation of IVIM DWI into everyday clinical practice.
Until recently, DWI performed in the body has been significantly more robust at 1.5 T compared with 3.0 T. Initial experience of the technique in oncology has been largely gained through developments at 1.5 T. However, recent hardware and software innovations have stimulated the development of body DWI at 3.0 T. This article considers the advantages and disadvantages of performing body DWI at 3.0 T, followed by a discussion of the technical implementation of body DWI at 3.0 T in oncology, including whole-body DWI. The current evidence for clinical applications of DWI at 3.0 T for oncologic evaluation is discussed. In addition, the potential of body DWI at 3.0 T combined within a PET-MR hybrid imaging system is highlighted. Knowledge of the basics of DWI and the implementation of the technique for body imaging at 1.5 T is assumed and so this is not discussed. The reader is referred to several review articles published on the subject.
Introduction
In the past few years, diffusion-weighted imaging (DWI) has become a standard imaging sequence for the evaluation of patients with cancer, especially those on 1.5-Tesla (T) magnetic resonance (MR) systems. This transformation in clinical practice has been brought about by improvements in MR hardware and software, as well as the growing clinical evidence for its use to detect disease, characterize lesions, and assess tumor response to treatment. Furthermore, because the technique is quick to perform, without the need for exogenous contrast administration, it can be routinely deployed for body MR imaging studies without significantly increasing the examination time.
In clinical practice, DWI is largely used as a qualitative tool, although it is a quantitative functional MR imaging technique. Imaging performed at higher diffusion weightings (typically at b-values of 750–1000 s/mm 2 in the body) effectively suppresses the signal from normal background tissue, thus enhancing the conspicuity of cellular disease, which remains high signal intensity. Such qualitative deployment of DWI is widely used in daily practice as a tool to improve tumor detection that may be missed on conventional or contrast-enhanced imaging sequences. However, quantitative DWI is likely to become more important in oncology because these measurements provide objective quantification of water mobility in tissues, which can be applied to aid tissue characterization and to evaluate tumor response to treatment. There is emerging evidence that quantitative DWI can also yield novel predictive or prognostic information.
The apparent diffusion coefficient (ADC), calculated using 2 or more b-values, remains the most widely used quantitative DWI parameter and has been shown to have good measurement repeatability within and between scanners, particularly at 1.5 T. However, there is widening recognition of the nonmonoexponential signal attenuation at increasing b-values in tissues. Consequently, there is now significant interest in applying nonmonoexponential diffusion models to derive additional quantitative parameters that inform on tissue water diffusivity. These nonmonoexponential diffusion models include intravoxel incoherent motion (IVIM), stretched exponential, and diffusion kurtosis models. To enable these models to be used with confidence, there is a need for meticulous image acquisition using more than 2 b-values, to ensure that images have good signal/noise ratio and to ascertain their measurement repeatability. For example, it has been shown that the perfusion-sensitive parameters of perfusion fraction (f) and pseudodiffusion coefficient (D*) have poor measurement repeatability, particularly in hypovascular lesions, which has limited wider translation of IVIM DWI into everyday clinical practice.
Until recently, DWI performed in the body has been significantly more robust at 1.5 T compared with 3.0 T. Initial experience of the technique in oncology has been largely gained through developments at 1.5 T. However, recent hardware and software innovations have stimulated the development of body DWI at 3.0 T. This article considers the advantages and disadvantages of performing body DWI at 3.0 T, followed by a discussion of the technical implementation of body DWI at 3.0 T in oncology, including whole-body DWI. The current evidence for clinical applications of DWI at 3.0 T for oncologic evaluation is discussed. In addition, the potential of body DWI at 3.0 T combined within a PET-MR hybrid imaging system is highlighted. Knowledge of the basics of DWI and the implementation of the technique for body imaging at 1.5 T is assumed and so this is not discussed. The reader is referred to several review articles published on the subject.
Body diffusion-weighted imaging at 3.0 Tesla: advantages and disadvantages
In theory, the measured MR signal is proportional to the square of the static magnetic field, suggesting that there should be a 4-fold increase in signal at 3.0 T compared with at 1.5 T. However, in practice, because of several factors, including changes in tissue relaxation rates and increased magnetic susceptibility in tissues at 3.0 T, the signal gain is usually of the order of 30% to 60%. Furthermore, image noise also scales linearly with the increase in field strength. Nonetheless, the increased image signal/noise at 3.0 T can be harnessed for reduced-field-of-view imaging over specific anatomic locations, such as the prostate, cervix, uterus, pancreas, and breast, as well as the head and neck regions; this can translate to higher spatial resolution DWI of these areas.
The main disadvantages of performing body DWI at 3.0 T are the increased sensitivity to susceptibility artifacts and the higher likelihood of chemical-shift artifacts from poor fat suppression as a consequence of B1 magnetic field inhomogeneity. However, there are now technical implementations that can help to overcome these limitations. Using the readout-segmented echo-planar imaging (EPI) technique, it is possible to reduce the degree of susceptibility artifacts and geometric distortion encountered. In addition, advances in hardware and software shimming (eg, using advanced or image-based shimming options) improves magnetic field homogeneity, leading to more uniform fat suppression across large fields of view, thus reducing the number of poor-quality DWI studies that are degraded by chemical-shift artifacts as a consequence of poor fat suppression. Dual (parallel) radiofrequency transmit systems have reduced the B1 inhomogeneity, which has improved fat suppression at 3.0 T.
Combinatorial chemical and fat-suppression techniques can also be harnessed to advantage at 3.0 T DWI because of the larger differences in the MR precessional frequencies between tissues. For example, when performing DWI in women with silicone breast implants, combining spectral fat suppression technique with a slice-selective gradient reversal scheme allows successful dual suppression of fat and silicone at 3.0 T, which is difficult to achieve at 1.5 T ( Fig. 1 ). In this regard, DWI of the breasts in women with silicone breast implants is more likely to yield meaningful results at 3.0 T compared with at 1.5 T.
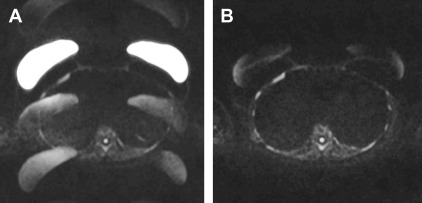
Technical implementation of body diffusion-weighted imaging at 3.0 Tesla
Modern state-of-the-art MR systems at 3.0 T have evolved considerably in the past few years with improvement in surface coil technologies, gradient performance, and advanced shimming options. This progress has led to an overall improvement in the quality of body DWI achievable at 3.0 T, including whole-body DWI (WB-DWI).
When performing body DWI at 3.0 T, the guiding principles applicable to imaging at 1.5 T remain important at 3.0 T. Sequence optimization to ensure good image signal/noise and to minimize associated artifacts are paramount in obtaining consistent high-quality images. Important considerations for the optimization of DWI acquisitions are summarized in Table 1 . In addition to these general measures, there are specific technical approaches that merit further discussions.
Imaging Parameter | Optimization for DWI |
---|---|
TE | Choose lowest possible TE to maximize signal/noise ratio |
Matrix size | Use small matrix size to improve image signal/noise |
Field of view | Large field of view improves image signal/noise |
Section thickness | Thick image section improves image signal/noise |
Fat suppression | Inversion-recovery fat-suppression technique more robust over large fields of view |
Diffusion gradient application scheme | Monopolar gradient ensures lowest TE values Bipolar gradients can help to reduce geometric distortions Simultaneous gradient application schemes (eg, 3 scan trace) technique helps to minimize TE |
b-Values | Choose b-values appropriate for the tissue of study Ensure sufficient signal averages for good image signal/noise at high b-values |
Receiver bandwidth | Optimized receiver bandwidth helps to reduce TE, geometric distortion, and chemical shift artifacts |
Magnetic Resonance Shimming
Shimming refers to the process of adjusting the homogeneity of the static MR magnetic field. On modern scanners, shimming is both passive and active. Passive shimming is achieved by placing pieces of steel around the superconducting magnetic such that these produce their own magnetic fields, which work in synergy with the main field to improve field homogeneity. Active shimming is effected by adjusting electrical currents through specific shim coils to generate corrective magnetic fields, thus overcoming local field inhomogeneity.
In the past few years, active shimming technologies have become more sophisticated, with advanced higher-order shimming options becoming available on many 3.0-T MR platforms. More recently, individualized image-based shimming has been successfully implemented, which makes use of the measured individual voxel signal intensities to effect field corrections. These more sophisticated shimming options are often more time consuming to perform, but can significantly improve the quality of DWI at 3.0 T. Consequently, it is now possible to obtain high-quality diffusion-weighted images, including multistation or whole-body images, using commercial 3.0-T systems ( Fig. 2 ). Station-to-station spinal misalignment, which used to be an issue with multistation body DWI at 3.0 T, can largely be averted when advanced shimming techniques are applied.
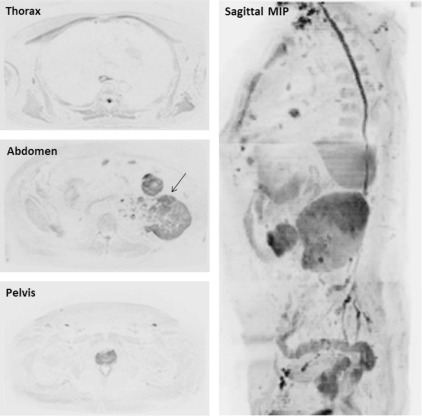
Reduced-field-of-view Imaging
The increased signal/noise at 3.0 T can be harnessed to obtain high-spatial-resolution diffusion-weighted images, by using smaller fields of view. Reduced-field–of-view DWI has been implemented on all the major MR vendor systems, which use inner-volume excitation, outer-volume signal suppression, or a combination of both techniques to enhance image quality. The technique has been harnessed to advantage, especially on parallel-transmit MR systems, in which two-dimensional–selective parallel-transmit excitation can be used to prescribe a selected field of view for evaluation.
Reduced-field–of-view diffusion-weighted MR techniques have been applied for the evaluation of the breast, pancreas, prostate, spinal cord, peripheral nerves, head/neck tumors, and the thyroid glands. By reducing the number of phase-encoding steps within the smaller imaged volume, it is possible to reduce both echo and scan time. Furthermore, the ability to use shorter echo times also means improved image signal/noise and reduced sensitivity to susceptibility artifacts. Harnessing the dual-source parallel-transmit radiofrequency excitation technique also improves B1 field homogeneity at 3.0 T, thus improving image quality and reducing artifacts.
Readout-segmented Echo-planar Imaging
Diffusion-weighted MR imaging based on readout-segmented EPI was introduced to reduce geometric distortions, image blurring, and artifacts. Using the readout-segmented technique allows reduction in the echo-train length and echo time, thus improving image blurring, and reducing susceptibility artifacts and geometric distortion. The technique also reduces the sensitivity to motion-induced phase errors. Using this technique allows higher spatial resolution for DWI in the body at field strengths of 3.0 T and beyond, and has been successfully applied for the evaluation of tumors in the breast, kidneys, and the pelvis ( Fig. 3 ).
One study compared the use of readout-segmented versus conventional single-shot EPI techniques for evaluating breast cancer, and found the readout-segmented technique to be superior for structure distinction, lesion delineation, reduction in ghosting artifact, and overall image quality. Another study compared readout-segmented with reduced-field-of-view DWI for the evaluation of breast cancers at 3.0 T. The image signal/noise, lesion conspicuity, and overall image quality were significantly higher for reduced-field-of-view EPI. Depending on the specific scan parameters used, it may be difficult to generalize these observations. Nonetheless, this study emphasizes the need to optimize image signal/noise when using either the readout-segmented or reduced-field-of-view techniques across the body.
For the evaluation of pelvic tumors at 3.0 T, geometric distortion, imaging blurring, ghosting artifacts, lesion conspicuity, and the overall image quality were significantly better using readout-segmented echo-planar DWI compared with conventional single-shot echo-planar acquisitions. Furthermore, the mean difference and the limits of agreement between the ADC values obtained from the two methods were 0.01 (−0.08, 0.10) × 10 −3 mm 2 /s, suggesting good concordance in the ADC quantification compared with the conventional EPI technique.
Clinical applications of body diffusion-weighted imaging for oncology at 3.0 Tesla
In general, body DWI is easier to implement at 1.5 T and most clinical applications in oncology can be addressed on 1.5-T MR systems. However, imaging at 3.0 T has continued to improve and high-quality diffusion-weighted images can now be reliably attained at 3.0 T; ADC quantification in the body has also been shown to be reliable and repeatable.
Disease Detection
One of the most widely used clinical application for DWI is for the detection of liver metastases. The application of a low–b-value diffusion-weighting effectively suppresses the signal from the intrahepatic vasculature, thus enhancing the detection of focal metastases. Although most liver MR studies are still performed at 1.5 T, imaging at 3.0 T is increasing. Many DWI studies at 1.5 T use a bipolar gradient scheme at image acquisition to optimize image quality. However, one study at 3.0 T found that imaging using a monopolar gradient scheme resulted in better image quality in the liver compared with a bipolar gradient. Nonetheless, imaging optimization at 3.0 T can be challenging and to a significant extent depends on the vendor platform, hardware, and software available. A recent study at 3.0 T showed the value of DWI to confidently diagnose pseudolesions, which are typically invisible on DWI, in patients with liver cirrhosis ( Fig. 4 ).
The anatomic site where 3-T imaging has been shown to be of significant advantage compared with 1.5 T is the prostate gland. There is compelling evidence for the use of MR imaging for the detection of prostate cancer in patients with increased serum prostate-specific antigen (PSA) levels, and to help localize disease for image-guided targeted biopsy. The higher signal/noise at 3.0 T allows diffusion-weighted images with higher spatial resolution (using reduced-field-of-view or readout-segmented EPI techniques) and also can be used for obtaining images with ultrahigh b-values (≥1000 s/mm 2 ).
Because of the T2 shine-through from the normal peripheral zone of the prostate gland, the endogenous contrast between tumor and the normal gland may be reduced (at b-values <1000 s/mm 2 ), making it more difficult to identify disease on these images. However, imaging at 3.0 T affords a higher signal/noise, which allows ultrahigh–b-value images of 1500 to 2000 s/mm 2 to be acquired, thus overcoming the T2 shine-through effects. One study showed that using a b-value of 2000 s/mm 2 improved cancer detection in the prostate gland in the peripheral zone, but was unchanged for the transitional zone. Where acquisition of ultrahigh b-value images may be impractical or noise limited, it may be possible to use computed ultrahigh–b-value images that are mathematically generated from images acquired at lower b-values (typically <1000 s/mm 2 ) to improve the diagnostic performance ( Fig. 5 ). Despite this high sensitivity of DWI for prostate cancer detection, lesions may still be missed. A recent study found that missed lesions at DWI using a b-value of 2000 s/mm 2 were typically small (mean diameter of 5 mm) but may contain a minor Gleason grade 4 component. In the postradiotherapy setting, DWI was superior to T2-weighted MR imaging for the detection of local disease recurrence in patients presenting with an increasing serum PSA level.
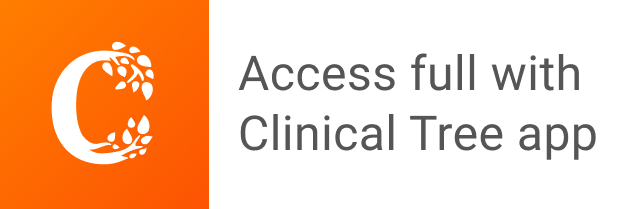