Breast MR imaging has increased in popularity over the past 2 decades due to evidence of its high sensitivity for cancer detection. Current clinical MR imaging approaches rely on the use of a dynamic contrast-enhanced acquisition that facilitates morphologic and semiquantitative kinetic assessments of breast lesions. The use of more functional and quantitative parameters holds promise to broaden the utility of MR imaging and improve its specificity. Because of wide variations in approaches for measuring these parameters and the considerable technical challenges, robust multicenter data supporting their routine use are not yet available, limiting current applications of many of these tools to research purposes.
Key points
- •
Current clinical breast MR imaging approaches focus on high morphologic detail and semiquantitative kinetic information that allow high sensitivity and moderate specificity for breast cancer detection.
- •
Advanced functional MR imaging parameters can improve the ability to assess biology in vivo using imaging correlates of vascularity, cellularity, and chemical composition of breast lesions.
- •
Preliminary findings support the use of a functional, multiparametric breast approach to improve breast MR imaging specificity and the biological characterization of breast cancer.
- •
Currently, technical challenges and a lack of standardization in approach limit applicability of many functional breast MR imaging techniques, which should be addressed with future research.
Introduction
Breast cancer is extremely common, striking 1 in 8 American women, and is the second leading cause of cancer death among women in the United States. Breast cancer mortality has decreased substantially over the past several decades, owing both to earlier-stage breast cancer detection through improved imaging techniques and improved therapeutics. Although breast MR imaging is a recent imaging technique, it was first proposed to aid early breast cancer detection in the 1970s, which was supported by the discovery that abnormal breast tissue demonstrates differences in longitudinal (T1) and transverse (T2) relaxation times in vitro compared with normal tissue. It was not until the elucidation, however, that most breast cancers demonstrate higher signal on T1-weighted images after the administration of gadolinium-based contrast material that breast MR imaging became a widely used tool for in vivo characterization of breast cancer. Although optimal evidence-based uses continue to evolve, common clinical indications for conventional contrast-enhanced breast MR imaging currently include supplemental screening for high-risk women, preoperative evaluation of breast cancer extent of disease, assessment of equivocal findings on standard imaging and/or clinical examination, and evaluation of breast cancer response to neoadjuvant chemotherapy.
Perhaps the greatest driving force behind the increasingly wide adoption of breast MR imaging at many centers is its exquisite sensitivity (reported to approach 100%) for breast cancer detection. Multiple studies have shown that conventional contrast-enhanced breast MR imaging has the highest sensitivity of any imaging modality for breast cancer detection in asymptomatic high-risk women and mammographically and clinically occult additional disease in the contralateral and ipsilateral breast in patients with recently diagnosed breast cancer. A major barrier to wider adoption of this technique for average and intermediate risk patients, however, is its modest specificity due to overlap in the imaging features of benign and malignant lesions, with wide variations in the positive predictive value of breast MR imaging reported in the literature (24%–89%).
MR imaging also has been increasingly been studied as a tool to determine which newly diagnosed breast cancers are likely to respond to presurgical, or neoadjuvant, chemotherapy. Several early studies examining the use of MR imaging to assess early response to neoadjuvant chemotherapy found that changes in size or volume and enhancement kinetic profiles on MR imaging were associated with favorable responses to therapy, including pathologic complete response (pCR). These findings suggested that MR imaging could be used to optimize medical therapy regimens for each individual. The general superiority of MR imaging over clinical examination and standard imaging techniques for predicting pCR was suggested in multiple single-center studies and confirmed in a large multicenter trial. Although standard contrast-enhanced MR imaging is the most accurate modality for predicting important neoadjuvant therapy outcomes, its clinical impact has been limited by its cost and modest overall performance in this setting.
Although MR imaging is commonly used at many centers to further evaluate equivocally suspicious clinical or imaging findings, several obstacles have limited application of MR imaging in this clinical setting. Barriers to cost-effective implementation of MR imaging to reduce unnecessary biopsies prompted by conventional imaging or clinical examination include the low cost associated with image-guided biopsies, which are highly accurate and safe compared with serial imaging, and the high negative predictive value (NPV) (approximately 98%) needed to obviate the biopsy of a suspicious finding based on current American College of Radiology (ACR) Breast Imaging-Reporting and Data System (BI-RADS) guidelines. Early studies have demonstrated that the NPV of MR imaging for this clinical indication ranges from 76% for further evaluation of suspicious mammographic calcifications to 85% in the setting of any suspicious mammographic or clinical finding. Studies from the authors’ institution found that although breast MR imaging has high sensitivity and high NPV for this clinical indication, the added breast cancer yield was too low to routinely recommend breast MR imaging for problem solving. More recently, Strobel and colleagues found that the use of conventional breast MR imaging to evaluate suspicious (BI-RADS category 4) findings identified on screening mammography or ultrasound could help avoid up to 92% of unnecessary biopsies and was particularly accurate in evaluating lesions that were not comprised entirely of calcifications.
The basis for the strong clinical performance of conventional breast MR imaging has been through utilization of an imaging approach that emphasizes anatomic and morphologic detail through high spatial resolution and limited temporal resolution dynamic contrast-enhanced (DCE) technique (DCE–MR imaging). This approach provides limited insight on physiologic features of breast tissue and pathology through the measurement of semiquantitative kinetic features. Although these basic kinetic enhancement features, including peak initial-phase and delayed-phase curve descriptors, have shown some ability to distinguish between malignant and benign lesions, they in general have provided a small incremental value to standard morphologic descriptions. As a result, there is increasing interest in exploring whether multiparametric approaches to breast MR imaging that incorporate more highly quantitative pharmacokinetic DCE–MR imaging modeling and other functional MR imaging techniques, such as diffusion-weighted imaging (DWI) and magnetic resonance spectroscopy (MRS), to probe specific biological properties, such as abnormal vessel permeability, cellularity, and chemical composition, can further advance the use of MR imaging for these specific clinical settings.
Introduction
Breast cancer is extremely common, striking 1 in 8 American women, and is the second leading cause of cancer death among women in the United States. Breast cancer mortality has decreased substantially over the past several decades, owing both to earlier-stage breast cancer detection through improved imaging techniques and improved therapeutics. Although breast MR imaging is a recent imaging technique, it was first proposed to aid early breast cancer detection in the 1970s, which was supported by the discovery that abnormal breast tissue demonstrates differences in longitudinal (T1) and transverse (T2) relaxation times in vitro compared with normal tissue. It was not until the elucidation, however, that most breast cancers demonstrate higher signal on T1-weighted images after the administration of gadolinium-based contrast material that breast MR imaging became a widely used tool for in vivo characterization of breast cancer. Although optimal evidence-based uses continue to evolve, common clinical indications for conventional contrast-enhanced breast MR imaging currently include supplemental screening for high-risk women, preoperative evaluation of breast cancer extent of disease, assessment of equivocal findings on standard imaging and/or clinical examination, and evaluation of breast cancer response to neoadjuvant chemotherapy.
Perhaps the greatest driving force behind the increasingly wide adoption of breast MR imaging at many centers is its exquisite sensitivity (reported to approach 100%) for breast cancer detection. Multiple studies have shown that conventional contrast-enhanced breast MR imaging has the highest sensitivity of any imaging modality for breast cancer detection in asymptomatic high-risk women and mammographically and clinically occult additional disease in the contralateral and ipsilateral breast in patients with recently diagnosed breast cancer. A major barrier to wider adoption of this technique for average and intermediate risk patients, however, is its modest specificity due to overlap in the imaging features of benign and malignant lesions, with wide variations in the positive predictive value of breast MR imaging reported in the literature (24%–89%).
MR imaging also has been increasingly been studied as a tool to determine which newly diagnosed breast cancers are likely to respond to presurgical, or neoadjuvant, chemotherapy. Several early studies examining the use of MR imaging to assess early response to neoadjuvant chemotherapy found that changes in size or volume and enhancement kinetic profiles on MR imaging were associated with favorable responses to therapy, including pathologic complete response (pCR). These findings suggested that MR imaging could be used to optimize medical therapy regimens for each individual. The general superiority of MR imaging over clinical examination and standard imaging techniques for predicting pCR was suggested in multiple single-center studies and confirmed in a large multicenter trial. Although standard contrast-enhanced MR imaging is the most accurate modality for predicting important neoadjuvant therapy outcomes, its clinical impact has been limited by its cost and modest overall performance in this setting.
Although MR imaging is commonly used at many centers to further evaluate equivocally suspicious clinical or imaging findings, several obstacles have limited application of MR imaging in this clinical setting. Barriers to cost-effective implementation of MR imaging to reduce unnecessary biopsies prompted by conventional imaging or clinical examination include the low cost associated with image-guided biopsies, which are highly accurate and safe compared with serial imaging, and the high negative predictive value (NPV) (approximately 98%) needed to obviate the biopsy of a suspicious finding based on current American College of Radiology (ACR) Breast Imaging-Reporting and Data System (BI-RADS) guidelines. Early studies have demonstrated that the NPV of MR imaging for this clinical indication ranges from 76% for further evaluation of suspicious mammographic calcifications to 85% in the setting of any suspicious mammographic or clinical finding. Studies from the authors’ institution found that although breast MR imaging has high sensitivity and high NPV for this clinical indication, the added breast cancer yield was too low to routinely recommend breast MR imaging for problem solving. More recently, Strobel and colleagues found that the use of conventional breast MR imaging to evaluate suspicious (BI-RADS category 4) findings identified on screening mammography or ultrasound could help avoid up to 92% of unnecessary biopsies and was particularly accurate in evaluating lesions that were not comprised entirely of calcifications.
The basis for the strong clinical performance of conventional breast MR imaging has been through utilization of an imaging approach that emphasizes anatomic and morphologic detail through high spatial resolution and limited temporal resolution dynamic contrast-enhanced (DCE) technique (DCE–MR imaging). This approach provides limited insight on physiologic features of breast tissue and pathology through the measurement of semiquantitative kinetic features. Although these basic kinetic enhancement features, including peak initial-phase and delayed-phase curve descriptors, have shown some ability to distinguish between malignant and benign lesions, they in general have provided a small incremental value to standard morphologic descriptions. As a result, there is increasing interest in exploring whether multiparametric approaches to breast MR imaging that incorporate more highly quantitative pharmacokinetic DCE–MR imaging modeling and other functional MR imaging techniques, such as diffusion-weighted imaging (DWI) and magnetic resonance spectroscopy (MRS), to probe specific biological properties, such as abnormal vessel permeability, cellularity, and chemical composition, can further advance the use of MR imaging for these specific clinical settings.
Breast MR imaging parameters
There is no single way to achieve high-quality breast MR imaging, and this is particularly true when considering a multiparametric approach to MR imaging acquisition. Nonetheless, MR imaging should include an acquisition using a high-field (B 0 ) magnet (≥1.5T) that is bilateral with complete breast and axilla coverage. Only dedicated breast surface coils are appropriate for breast MR imaging applications, because the built-in body coil cannot provide enough signal-to-noise (SNR) for high-quality breast imaging.
Dynamic Contrast-Enhanced MR Imaging
Currently, a DCE–MR imaging acquisition is central to breast MR imaging protocols, and the ACR Breast MRI Accreditation Program (BMRAP) mandates that a multiphase T1-weighted acquisition be performed for clinical breast MR imaging. Due to time and technical restraints, DCE–MR imaging protocols generally emphasize either high spatial resolution and full coverage or high temporal resolution in imaging of contrast uptake kinetics in breast. More sophisticated acquisition strategies, however, that undersample the periphery of k-space on subsequent dynamic acquisitions and more frequently sample the center of k-space can provide simultaneous high spatial, high temporal resolution acquisitions with full bilateral coverage ( Fig. 1 ). There is wide variation among centers on the number of postcontrast phases acquired. The ACR BMRAP requires acquisition of a minimum of 2 postcontrast T1-weighted images, with the first postcontrast sequence acquired within 4 minutes of contrast injection. DCE–MR imaging acquisition provides 2 primary imaging features that can be used to evaluate breast lesions: morphology and kinetic enhancement characteristics.
Morphology
There are 3 general morphologic descriptors of enhancing findings of the breast, which are defined by the ACR BI-RADS atlas: foci, masses, and nonmass enhancements (NMEs). Masses and NMEs also have additional descriptors that can further refine an interpreting radiologist’s suspicion for malignancy. Prior studies have shown that morphologic features are perhaps the most important factor for initial assessment of likelihood of malignancy of a given lesion. Suspicious morphologic features for masses include irregular shape, heterogeneous or rim internal enhancement, and irregular or spiculated margins. Suspicious NME morphologic features include segmental or linear distribution with heterogeneous or clustered ring enhancement. Breast cancers that present as NME are more likely to reflect in situ carcinoma than masses; however, significant overlap exists in morphologic presentation on MR imaging of invasive breast cancer, ductal carcinoma in situ, and atypical/high-risk lesions.
Dynamic contrast-enhanced kinetic enhancement features
Differential enhancement of malignancies relative to normal breast tissue is based on the fact that malignancies typically recruit abnormal new blood vessels to support their growth (ie, angiogenesis). The rate at which these abnormal vessels allow nutrients to spill into a tumor and the rate cell cycle byproducts are removed from a tumor can be characterized through assessing kinetic enhancement curves obtained from DCE–MR imaging. Most commonly, breast kinetic enhancement features are measured semiquantitatively using modest temporal resolution with at least 2 to 3 postcontrast T1-weighted acquisitions, with k-space centered at approximately 90 to 120 seconds after contrast injection for the first postcontrast images. Using the data obtained at each of these time points, a time-signal intensity curve can be determined for a given lesion or region of interest (ROI), allowing assessment of 2 phases of enhancement: initial phase, within approximately 2 minutes of contrast injection, and late (or delayed) phase, after 2 minutes or after peak enhancement ( Fig. 2 ). In the initial phase, enhancement classifications of slow, medium, and fast are determined by signal intensity increase ( SI %increase ) defined by the equation:
where SI pre is the baseline signal intensity of an ROI and SI pos t is the signal intensity of the same ROI after contrast injection.
In the delayed phase, enhancement curves can be classified by 3 basic curve types: persistent, plateau, and washout. Persistent delayed enhancement is generally considered a benign enhancement curve type, whereas plateau delayed enhancement is of intermediate suspicion for malignancy, and washout delayed enhancement is the most suggestive of malignancy. Although the most classic combined curve type for malignant breast lesions is fast initial enhancement followed by early washout (sometimes referred to as a type III curve ), there is significant overlap of semiquantitative kinetic curve types among benign and malignant lesions.
MR imaging techniques that acquire postcontrast images with high temporal resolution can allow for more elegant assessment of contrast kinetics through pharmacokinetic modeling techniques. Pharmacokinetic models enable quantitative assessments of contrast agent exchange between the intravascular and the interstitial space, providing measures related to tumor blood flow, microvasculature, and capillary permeability. A 2-compartment model is the most commonly used approach, measuring the exchange of contrast between tissue (in this case breast tissue) and the plasma space and was first proposed in an MR imaging context by Tofts and Kermode. The concentrations of the gadolinium tracer for each compartment vary with time after the bolus injection of the contrast agent, and quantitative metrics can be measured by this model using the following relationship:
where the volume transfer constant K trans reflects the rate of transfer of gadolinium from plasma to the tissue (unit: min −1 ) (see Fig. 1 D); the transfer rate constant k ep (min −1 ) reflects the reflux of contrast agent from the extravascular extracellular space to the plasma compartment; and v e (%) reflects the leakage of fractional volume from the extravascular extracellular space into the plasma compartment.
Promising clinical applications of quantitative dynamic contrast-enhanced MR imaging
Several investigators have demonstrated that some of these pharmacokinetic parameters, in particular K trans and k ep , hold value for improved discrimination of malignant from benign breast pathologies and may even be used as biomarkers of disease subtypes. Li and colleagues demonstrated that K trans and k ep values progressively increased when measuring normal glands, benign lesions, and malignant lesions, noting that invasive ductal carcinomas and ductal carcinoma in situ lesions exhibited significantly higher K trans and k ep values than ductal dysplasias. Huang and colleagues demonstrated that using K trans values of lesions found suspicious on standard clinical breast MR imaging, a potential cutoff value could be used such that lesions with lower K trans values could avoid biopsy and thereby decrease false-positive MR examinations. Finally, DCE–MR imaging holds potential for assessing alterations in tumor perfusion in response to preoperative therapies ( Fig. 3 ). K trans values have been shown in a recent meta-analysis to be among the most promising MR imaging parameters for prediction of near-pCR to neoadjuvant chemotherapy, outperforming standard tumor size measurements.
Technical challenges and considerations of quantitative dynamic contrast-enhanced MR imaging
To perform DCE–MR imaging pharmacokinetic modeling and calculate quantitative parameters, knowledge of both the precontrast T1 relaxation times of the tumor or tissue being imaged and the arterial input function (AIF), or the concentration of contrast agent as it changes over time within the arterial blood, is required. Measuring each of these parameters introduces unique challenges and potential for error. Precontrast T1 mapping is an essential step to convert DCE–MR imaging signal intensity into contrast agent concentration. T1 mapping requires acquisition of an additional series of images prior to DCE–MR imaging, most commonly using varying flip angle or inversion recovery approaches, and thus adds to the overall examination times. Moreover, variable flip angle approaches are prone to inaccuracies due to B1 inhomogeneity, a common issue for breast imaging, particularly at higher field strengths. Most models require that the AIF be measured directly for each subject, which is often challenging to perform and necessitates acquisition tradeoffs (in coverage and/or spatial resolution) to achieve the very high temporal resolution required to accurately sample the rapidly changing AIF. Furthermore, AIF measures can be sensitive to patient motion between dynamic acquisitions. One common approach to avoid the challenge of directly calculating the AIF is to use an average AIF calculated from a larger population for whom the injection site, dose, and rate were kept constant. Yankeelov and colleagues have proposed another method to circumvent this problem by estimating the AIF using a reference region model and found that such an approach correlated well with direct AIF measurement. Novel high spatiotemporal DCE–MR imaging acquisition strategies, as described previously, hold potential to provide high temporal resolution sampling of contrast enhancement curves without undesirable tradeoffs in spatial resolution and coverage, which may improve feasibility for utilization of pharmacokinetic analysis in clinical breast applications.
Theoretically, model-based pharmacokinetic parameters have the advantage over semiquantitative enhancement curve assessments of being objective measures of underlying physiology that are not affected by variability in scan parameters. Given the different modeling algorithms, multiple challenges, and varying potential solutions, however, each of which can create significant differences in quantitative measurements, the generalizability of individual studies of advanced pharmacokinetic parameters will require standardization of the technical approach and multicenter testing.
Diffusion-Weighted MR Imaging
DWI is a noncontrast MR imaging technique that measures the mobility of water molecules in vivo and probes tissue organization at the microscopic level. This water movement due to molecular diffusion, called brownian motion, is random in pure water. The motion of water molecules in vivo, however, is restricted by hindrances within intracellular and extracellular compartments. As a result, DWI reflects the microscopic cellular environment and is sensitive to biophysical characteristics, such as cell density, membrane integrity, and microstructure.
DWI has shown promise for the detection and characterization of breast cancer. Numerous studies have shown that malignant breast lesions exhibit decreased water diffusion, attributed primarily to the increased cell density associated with breast tumors. DWI is a short scan available on most commercial MR scanners that does not require any exogenous contrast and can be added to breast MR imaging examinations to provide additional unique information on tissue microstructural properties.
Apparent diffusion coefficient calculation
DWI uses motion-sensitizing gradients during MR image acquisition to probe local diffusion characteristics. The diffusion-weighted MR imaging signal is reduced in intensity proportional to the water mobility and is commonly described by the monoexponential equation:
where S 0 is the signal intensity without diffusion weighting, S D is the signal intensity with diffusion weighting, b is the applied diffusion sensitization (s/mm 2 ), and ADC is the apparent diffusion coefficient, defined as the average area a water molecule occupies per unit time (mm 2 /s). In general, ADC can be calculated directly from a minimum of 2 acquisitions with different diffusion sensitizations ( b-values ) using:
where b 1 is the minimum b -value (eg, 0 s/mm 2 ) and b 2 is the maximum b -value (eg, 800 s/mm 2 ); S 1 is the signal intensity at b = b 1 , S 2 is the signal intensity at b = b 2 , and repetition time and echo time remain constant. Due to restricted diffusion, breast malignancies commonly exhibit hyperintensity on DWI and lower ADC relative to normal breast parenchyma ( Fig. 4 ).
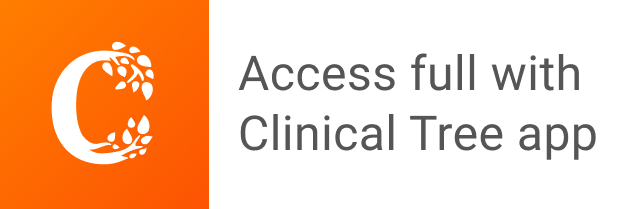