Introduction
The first reported in vivo localized proton ( 1 H) magnetic resonance spectroscopy (H1-MRS) studies of the human brain were first reported over 20 years ago. Since these early studies, in vivo H1-MRS brain studies have been shown to be a powerful technique to noninvasively investigate the biochemistry of the human brain and to assess in situ the neurochemical profile of brain tissue. Thus they can provide biomarkers of neurologic disorders even in cases where lesions are not seen in conventional anatomic MRIs. The noninvasive quality of H1-MRS and the spatially localized MR techniques that have been developed to examine in vivo the neurochemical profile of brain tissue makes them suitable not only for diagnostic purposes but also longitudinal follow-up studies. They have proven to be important tools at many institutions for the noninvasive clinical assessment of numerous neurologic disorders such as brain tumors, epilepsy, white matter disease processes, metabolic disorders, and brain trauma. However, even though proton MRS has been incorporated into clinical protocols and is accepted at many institutions worldwide, it is still considered an investigational technique by some healthcare organizations, who indicate that at the present time there has been “no large multicenter trial published demonstrating any added benefit of MRS over MRI in diagnosing or monitoring pathological processes such as brain tumors, and no clinical trials demonstrating improved outcomes evaluated with MRS alone compared to patients evaluated with conventional imaging modalities have been reported.” What is not understood by these healthcare organizations is that MRS and MRI are not competitive techniques but rather complementary techniques that can “add value” to the MR diagnostic procedure and lead to better patient management decisions. MRS provides information about the metabolic profiles of lesions, whereas conventional MRI provides anatomic profiles, and in most cases it should not be used alone in making clinical diagnostic decisions but used in conjunction with the clinical history of the patient and information from conventional anatomic and advanced MRI techniques. As an example, Moeller-Hartmann and coworkers examined the diagnostic benefits of adding MRS to a conventional anatomic MRI study of intracranial mass lesions (i.e., infarctions, primary brain tumors, metastatic lesions, primitive neuroectodermal tumors, abscesses). In this study they found that with conventional MRI alone, 96 out of 176 correct diagnoses were made (55.1%). Adding H1-MRS information to the MRI findings led to an increase in the number of correct diagnoses from 96 to 124 out of the 176 cases (a 15.4% increase in correct diagnoses). By adding MRS to the MRI study, the number of incorrect diagnoses decreased from 27 (15.3%) for MRI alone to 16 (9.1%). Similarly, in a 2012 study it was shown that H1-MRS added value to conventional MRI information in the preoperative characterization of the type and grade of brain tumors. This study found that information obtained from “H1-MRS significantly improved the radiologists’ MRI based characterization of grade IV tumors (glioblastomas (GBs), metastases, medulloblastomas, and lymphomas) in the cohort” (area under the receiver operating curve [AUC] from 0.85 in the MRI [alone] to 0.93 in the MRI plus MRS reevaluation [MRI plus MRS] and also in the less malignant glial tumors [AUC in the MRI reevaluation was 0.93 versus 0.81 in the MRI alone]).
This chapter will not review the basic principles of MRS and in vivo H1-MRS/MRSI of the human brain. The fundamental principles of this technique have been presented in detail elsewhere. The main body of this chapter will concentrate on the complementary use of H1-MRS with advanced MRI techniques (i.e., diffusion-weighted imaging [DWI], perfusion-weighted imaging [PWI], and permeability), and conventional MRI to improve the accuracy of diagnosis and delineation of brain tumors and in therapeutic decision making. The major reason for this is because of the important findings and recommendations on the use of MRS recently published by the MR Spectroscopy Consensus Group. This group was formed in 2011 under the auspices of the International Society of Magnetic Resonance in Medicine and is composed of leading imaging scientists, neuroradiologists, neurologists, oncologists, and clinical neuroscientists from universities, as well as vendors from the United States, Europe, and Asia. This international group was charged with the task of documenting “the impact of H-1 MR spectroscopy in the clinical evaluation of the central nervous system.” One of the major conclusions and recommendations of this group was that “MR spectroscopy adds diagnostic and prognostic benefits to MR imaging and aids in treatment planning and monitoring of brain cancers,” and that “clinical imaging centers specializing in combined use of MR imaging and spectroscopy should be established in all major clinical neurologic centers that offer standardized MR spectroscopy procedures for improved patient management.” This conclusion was based on the substantial body of both basic science and clinical MRS research that has been performed over the past 2 decades worldwide, with consistent results found across laboratories. This chapter will demonstrate that MRS, especially if combined with advanced MRI techniques, (1) can improve the diagnostic accuracy identifying neoplastic from nonneoplastic brain processes, (2) can identify the presence or absence of specific metabolites in the 1 H MR spectrum that can be used as biomarkers to identify various tumor processes, and (3) that the combination of conventional and advanced MRI and MRS can reduce the need for more invasive diagnostic procedures to assess the cellular characteristics of untreated and treated brain tumor processes.
The MR paradigms described in the brain tumor studies can serve as models for the potential development of combined MRS and MRI techniques to increase diagnostic accuracy and modify patient treatment planning to improve the therapeutic outcomes in other central system disorders presented in this chapter and in the MR Spectroscopy Consensus Group report.
MRI vs. MRS
There is no difference between the physical principals of MRI and MRS; both techniques are governed by the same principles of magnetism. MRI and MRS differ only in the manner in which the data obtained are analyzed and the type of information provided. In the case of MRI, data collected are analyzed in the time domain (namely, free induction decay signal; signal intensity vs. time) to obtain relaxation time (TR) information (namely, T1 [spin-lattice] and T2 [spin-spin]) of the nuclei. The data from the time domain information is then used to generate an anatomic image. In MRS, the time domain information is converted to the frequency domain (signal intensity vs. frequency) via Fourier transformation of the free induction decay time domain signal. The frequency information generated via Fourier transformation of the time domain signal is used to form a distribution of the intensities of chemical groups associated with various metabolites versus their Larmor resonance frequencies ( Fig. 17-1 ) to give a spectral profile of the metabolites within this region of the anatomic image. Thus the two MR techniques (i.e., conventional MRI vs. MRS) give different but complementary information about the region being examined. In the case of conventional MRI (namely, T1- and T2-weighted MRI), the information is mainly anatomic in nature, generated via water proton interactions with the tissues, whereas MRS gives information about the biochemical/metabolic profile of the anatomic region being examined. Advanced MRI techniques such as PWI and DWI give physiologic information about the vascularity and cellularity of the anatomic region being evaluated. This information is also complementary to the information obtained from conventional anatomic MRI studies and biochemical/metabolic MRS studies. The major advantage of employing MR techniques to assess a lesion is that these complementary diagnostic techniques are noninvasive and can be performed during the same examination session (normally < 1.5 hours) to obtain multiparametric diagnostic information.
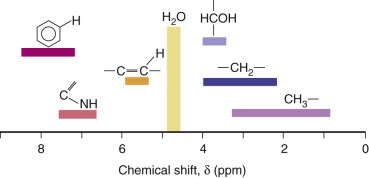
Evaluation of Brain Metabolites
MRS Observable In Vivo Brain Metabolites
Several important metabolites are evaluated in long echo time (TE) (135-288 milliseconds) proton MR spectra ( Fig. 17-2 ):
- •
N -acetylaspartate (NAA)
- •
Choline (Cho)
- •
Creatine/phosphocreatine (Cr/PCr)
- •
Lactate (Lac)
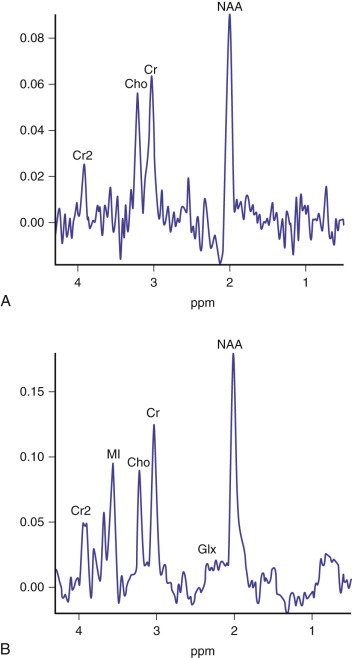
When short TEs (20-30 milliseconds) are used, a greater number of metabolites can be identified in the MR spectra; in addition to NAA, Cho, Cr, and Lac, the following may be identified :
- •
Glutamate (Glu)
- •
Glutamine (Gln)
- •
γ-Aminobutyric acid (GABA)
- •
Myoinositol (MI)
- •
Alanine (Ala)
- •
Glucose (Gc)
- •
Lipids and proteins
- •
Scylloinositol/taurine
Although it may appear advantageous to obtain spectra routinely at only short TEs to distinguish among different clinical entities, some disadvantages of short TE studies exist. For example, short TE spectra display greater baseline distortion, and estimating signal areas calls for more sophisticated processing software algorithms. However, to maximize metabolite information, both a short and long TE MRS study should be performed.
N -Acetylaspartate.
NAA accounts for the majority of the NAA resonance at 2.01 ppm; this signal is the most prominent one in normal adult brain proton MRS and is used as a reference for determination of chemical shift. The NAA signal also contains contributions from other N -acetyl groups, such as N -acetylaspartyl glutamate (NAAG), N -acetylated glycoproteins, and amino acid residues in peptides. NAA is second only to Glu as the most abundant free amino acid in the normal adult brain. The function of this amino acid is not fully understood despite its early discovery in 1956 by Tallan.
From animal studies, NAA is believed to be involved in coenzyme A (CoA) interactions and in lipogenesis within the brain. Specifically, such studies suggest that NAA is synthesized in the mitochondria from aspartate and acetyl CoA and transported into the cytosol where it is converted by aspartoacylase into aspartate and acetate. Although NAA is widely regarded as a nonspecific neuronal marker, it has also been detected in immature oligodendrocytes and astrocyte progenitor cells.
Normal absolute concentrations of NAA in the adult brain are generally in the range of 8 to 9 mmol/kg, although regional and age-related variations in NAA concentration have been noted by Kreis and others. In normal adults, NAA concentrations in cortical gray matter are higher than those in white matter; in infants the concentrations in gray and white matter are similar (highly active lipid synthesis in immature white matter accounts for this difference from the adult pattern). NAA concentrations are decreased in many brain disorders, resulting in neuronal and/or axonal loss, such as in neurodegenerative diseases, stroke, brain tumors, epilepsy, and multiple sclerosis, but are increased in Canavan’s disease.
Creatine.
The main Cr signal is present at 3.03 ppm and demonstrates major contributions from methyl protons of creatine and phosphocreatine as well as minor contributions from GABA, lysine, and glutathione. A second, usually smaller Cr signal is seen at 3.94 ppm. Cr is probably involved in maintenance of energy-dependent systems in brain cells by serving as a reserve for high-energy phosphates in neurons and as a buffer in cellular adenosine triphosphate/diphosphate (ATP-ADP) reservoirs. Thus the Cr signal is an indirect indicator of brain intracellular energy stores.
The Cr signal is often used as an internal reference standard for characterizing other metabolite signal intensities because it tends to be relatively constant in each tissue type in normal brain; however, this is not always true in abnormal brain tissue, particularly in areas of necrosis. Cr concentrations in the brain are relatively high, with progressive increases noted from white matter to gray matter to cerebellum. Kreis and coworkers noted a mean absolute Cr concentration in normal adult brains of 7.49 ± 0.12 mmol/kg on the basis of a sample of 10 normal subjects, whereas Michaelis and colleagues reported a similar value of 5.3 mmol/kg. Total Cr values tend to be abnormally reduced in brain tumors, particularly malignant ones.
Choline.
The Cho resonance is present at 3.2 ppm and is attributable to trimethyl ammonium residues of free Cho as well as phosphocholine, phosphatidylcholine, and glycerophosphocholine. This signal reflects cell membrane synthesis and degradation. Thus all processes resulting in hypercellularity (e.g., primary brain neoplasms or gliosis) or myelin breakdown (demyelinating diseases) lead to locally increased Cho concentration, whereas hypomyelinating diseases result in decreased Cho levels. Kreis and coworkers have reported a mean absolute Cho concentration in normal adult brain tissue of 1.32 ± 0.07 mmol/kg. Michaelis and others have reported a similar value of 1.6 mmol/kg.
Myoinositol.
MI produces two signals noted at 3.56 ppm and 4.06 ppm. MI is the major component of the signal at 3.56 ppm, although contributions from MI-monophosphate and glycine are also present. MI is believed to be a glial marker because it is present primarily in glial cells and is absent in neurons.
A role in osmotic regulation of the brain has been attributed to MI. In addition, MI may represent both a storage pool for membrane phosphoinositides involved in synaptic transmission and a precursor of glucuronic acid, which is involved in cellular detoxification. A derivative, MI-1,4,5-triphosphate, may act as a second messenger of intracellular calcium-mobilizing hormones.
The mean absolute concentration of MI in normal brain tissues obtained in the Kreis series was 6.56 ± 0.43 mmol/kg. MI concentrations are abnormally increased in patients with demyelinating diseases, Alzheimer’s disease, and low-grade brain tumors.
Lactate.
Lac resonance is identified as a doublet (splitting into two distinct resonant signals separated by 7 Hz), produced by magnetic field interactions among adjacent protons (referred to as J-coupling ) centered at 1.32 ppm. A second Lac signal is present at 4.1 ppm but tends to be inconspicuous on spectra obtained with water suppression owing to its proximity to the water signal. Because Lac levels in normal brain tissue are absent or extremely low (<0.5 mmol/L), they are essentially undetectable on normal spectra. The presence of a visible Lac signal constitutes a nonspecific indicator of cellular anaerobic glycolysis, which may be seen with brain neoplasms, infarcts, hypoxia, metabolic disorders, or seizures. Lac may also accumulate within cysts or foci of necrosis.
Changing the TE using a point-resolved spectroscopy (PRESS) sequence enables confirmation of the presence of an abnormal Lac doublet and differentiation from lipid signals; at TE = 20 and 272 milliseconds the Lac doublet projects above baseline, and at TE = 136 milliseconds it is inverted below the baseline. In encephalopathic neonates, however, a doublet very similar to Lac may be seen at 1.15 ppm, which corresponds to propan-1,2-diol and may easily be mistaken for Lac; propan-1,2-diol is a solvent commonly used for administration of anticonvulsant medications to neonates.
Glutamate, Glutamine, and GABA.
The glutamate and glutamine (“Glx”) and GABA signals are a complex set of resonances noted between 2.1 and 2.5 ppm and consist of Glu, Gln, and GABA components. Glu is an excitatory neurotransmitter involved in neuronal mitochondrial metabolism and is the most abundant amino acid in the human brain. Gln is involved in both cellular detoxification and regulation of neurotransmitter activities. GABA is a product of decarboxylation of Glu via glutamic acid decarboxylase and serves as an inhibitory neurotransmitter. Abnormalities in this signal complex have been noted in schizophrenia and epilepsy.
Lipids and Proteins.
Lipids produce multiple resonances, the most important of which are noted at 0.8 to 0.9 and 1.2 to 1.3 ppm because of methyl and methylene protons of fatty acids, respectively. Membrane lipids are not usually identified unless very short TEs are employed, because they have very short TRs and are not normally observed. Although artifactual accentuation of these signals may be seen with voxel contamination by adjacent subcutaneous fat, they can be eliminated by using outer volume suppression saturation pulses. High-grade gliomas (HGGs), meningiomas, demyelinating processes, necrotic foci, and inborn errors of metabolism can show the presence of lipids owing to the presence of lipid droplets within necrotic tumors, loosening of the lipid-rich myelin sheath, and breakdown of plasma membrane structures of cells.
Quantitation of Metabolite Levels
Currently, metabolite concentrations are most often presented as peak area ratios of the metabolite resonance relative to the peak area resonance of Cr at 3.03 ppm rather than absolute concentrations. Despite the success of this qualitative approach in defining changes in metabolite levels in disease processes, it has recently been suggested that relative quantitation may introduce substantial errors and lead to erroneous metabolite values.
Alterations in the peak area ratios do not necessarily reflect a change in the metabolite; these can be due to a change in the metabolite of interest or Cr levels or both, which will affect the ratio or possibly even change the TR behavior of the metabolites, which will also affect the ratio. This needs to be understood when interpreting changes in relative peak area ratios. If changes in the absolute metabolite concentrations are essential in the interpretation of the spectral data, then relevant methods to obtain absolute metabolite concentrations in either single-voxel or multivoxel spectroscopy studies have been described and are available and readily applied.
Clinical Applications of Proton MRS
Evaluation of Normal Brain Development and Regional Brain Differences
Although most neurons are formed during intrauterine life, proliferation of dendrites, astroglia, and oligodendroglia continues after birth. The processes of myelination and neuronal and dendritic development are associated with changes in various brain metabolites during infancy and early childhood, which are demonstrated by MRS.
MI and Cho form the most prominent signals in the newborn MR spectrum. The reason for the prominence of these two components in the infant spectrum is presumed to be the presence of active myelination. MRS may enable earlier detection of myelination abnormalities in infants than is possible with conventional MRI sequences, because changes in the Cho and NAA signals occur before white matter signal abnormalities on standard imaging sequences.
Age-related differences in metabolite levels were reported in both gray and white matter during brain development. NAA concentration, which is very low in the newborn brain, rapidly increases during the first few months of life and continues to increase until 2 to 3 years. Preterm infants were noted to have lower brain NAA levels than full-term infants. Cho levels in newborns are twice as high as in adults. After birth, Cho concentration exponentially decreases and reaches adult levels by approximately 2 to 3 years of age. The high MI levels decline rapidly in the first 3 to 4 months and decrease to adult levels by approximately 1 year of age. Cr levels rise to adolescent levels by 4 months of age, presumably as a result of increasing energy demands of the developing brain. Glu levels do not exhibit any development-related differences. The scylloinositol signal is high in newborns and decreases over time. Age-related differences in metabolite levels are less pronounced during childhood and adolescence, and the spectral pattern stabilizes during early adulthood. A meta-analysis evaluating literature data on metabolite levels in normal aging (in subjects > 60 years) reported decreased frontal NAA and increased parietal Cho and Cr with aging.
Lac signal is seen in preterm and small-for-gestational-age infants. However, in appropriate-for-gestational-age term infants, it is abnormal to find higher than trace amounts of Lac, particularly following the first few hours of life, and such abnormal amounts of Lac signify brain injury. In preterm infants, the levels of Lac decrease progressively until the age of 40 postconceptional weeks.
Significant regional heterogeneity in spectral patterns has been noted both in the developing brain and in mature brains. In the developing brain, proton MR spectra demonstrate that different parts of the brain mature at different rates and at different times and that the more metabolically mature areas demonstrate lower MI and higher NAA levels than the less mature regions of brain; specifically, the basal ganglia, perirolandic cortex, and visual cortex mature before areas such as the frontal white matter, prefrontal cortex, and temporal cortex. NAA/Cho ratios in the frontal region were reported to be lower than those in the parietal lobe at birth, with subsequent increase during the first 6 months of life. Lower NAA and higher Cho levels were detected in the allocortex compared to the isocortex in healthy children and adults.
Tedeschi and colleagues have noted significant regional variations in metabolite ratios in adults ; NAA/Cho and NAA/Cr ratios varied by more than a factor of 2 for different brain regions, with high levels of Cho and Cr and low levels of NAA found in the cerebellum. High Cho levels were also reported in insular cortex, thalamus, and centrum semiovale compared to parietal or occipital gray and white matter; high NAA levels were detected in the centrum semiovale. In the mesial temporal lobe, the anterior mesial temporal lobe was found to have higher Cho concentration than the middle and posterior mesial temporal lobe.
Evaluation of Primary Brain Tumors (Gliomas)
Many different types of brain tumors have been studied with MRS, and fairly consistent patterns of metabolic abnormalities have been described in both glial and nonglial tumors. Proton MRS allows reliable differentiation of tumor margins from adjacent brain edema, which is often not possible with gadolinium (Gd)-enhanced MRI, which may under- or overestimate tumor size in approximately 40% of cases.
A multitude of applications of proton MRS exist in the field of brain tumor diagnosis and management, and MRS is clearly playing an increasingly valuable clinical role that complements the roles of conventional structural MRI and other advanced MRI techniques such as PWI and diffusion tensor imaging (DTI).
Noninvasive Histologic Grading of Gliomas.
Although it is possible to clearly distinguish glial neoplasms from normal brain tissue by MRS, controversy exists regarding the reliability of MRS in distinguishing among different histologic grades of astrocytomas and other brain tumors. For example, Shimizu and associates demonstrated that through semiquantitation of MRS signal intensities as a ratio to that of an external reference, it was possible to predict tumor malignancy ; higher-grade brain tumors were associated with higher Cho/reference values and lower NAA/reference values in their series. This, in conjunction with findings from Tamiya’s group regarding high correlation between Cho/NAA and Cho/Cr ratios and cellular proliferative activity, provides substantial evidence that proton MRS may be useful in the prediction of tumor grade.
In a series involving children, linear discriminant analysis and proton MRS demonstrated an 83% success rate in establishing the correct diagnosis of histologic grade of brain tumors. In a series of 27 patients with biopsy-confirmed brain tumors, Meyerand and colleagues showed that the combination of Lac/water and Cho/water ratios obtained from regions of contrast-enhancing brain tumor (with normalization of each metabolite signal area to the area of the unsuppressed water signal) permitted differentiation of low-grade astrocytomas from anaplastic astrocytomas and GBs.
However, not all studies have confirmed the ability of proton MRS to differentiate between different tumor grades. For example, Burtscher and colleagues have described a series of 26 intracranial tumors in which MRS allowed differentiation of infiltrative processes from circumscribed lesions but did not allow differentiation of different types of lesions within each category. In a multicenter study involving 86 cases of glial tumors, Negendank and coauthors showed that each type and grade of tumor was a metabolically heterogeneous group with significant overlap in spectral NAA/Cr and Cho/Cr ratios with tumors in other categories; all tumors demonstrated abnormally decreased NAA/Cr and increased Cho/Cr ratios with respect to normal brain parenchyma. Some of the reasons for these findings in early studies (i.e., before the year 2000) regarding the inability of proton MRS to reliably differentiate various tumor grades have included inconsistencies in quality of spectroscopic data among different institutions, use of only long TE spectra, and use of only single-voxel technique; many of these problematic issues have been addressed in more recent studies.
Studies have suggested that advanced physiologic MRI methods in conjunction with MRS may be more accurate for determination of tumor grade than MRS or anatomic MRI alone. For example, Law and colleagues demonstrated that relative cerebral blood volume (rCBV) perfusion MRI combined with proton MRS metabolite ratios data improved both sensitivity and positive predictive value (PPV) for the determination of glioma grade over structural MRI alone. The combination of rCBV, Cho/Cr, and Cho/NAA resulted in sensitivity, specificity, PPV, and negative predictive value of 93.3%, 60%, 87.5%, and 75%, respectively, compared to 72.5%, 65%, 86.1%, and 44.1%, respectively, with conventional MRI alone. The low specificity was due in part to the fact that high Cho levels were found in some low-grade lesions.
El-Sherbeny and coworkers recently showed that inclusion of MI/Cr ratios in the MRS analysis in conjunction with the MRI information obtained from conventional and advanced MRI techniques added additional diagnostic value to the differentiation of the grade of the brain tumor. A number of studies have shown that MI/Cr peak area ratios over 0.6 are highly characteristic of low-grade gliomas. With the addition of MI/Cr ratios to MRS data, the sensitivity and specificity of the MRI/MRS paradigm employed to determine the grade of the lesion was 91% and 90.5%, respectively, compared to the study of Law et al., which found a 93.3% sensitivity and only a 60% specificity. The study of El-Sherbeny examined 93 patients with histologically confirmed brain tumors (31% low grade and 69% high grade).
More recently, Caulo and colleagues reported a quantitative multiparametric MRI evaluation of 110 patients with either low- or high-grade gliomas (rCBV, apparent diffusion coefficient [ADC]), MRS (Cho/Cr and Cho/NAA), and conventional MRI that incorporated the analysis of four heterogeneous MRI tumor regions. They found that taking into account the heterogeneous nature of the gliomas and combining the studies with both advanced and conventional MR techniques significantly improved the discrimination between low- and high-grade brain gliomas (sensitivity of 84.4%, specificity of 100%, and an AUC of 0.95).
A meta-analysis by Hollingworth and colleagues published in 2006 explored the overall clinical utility of MRS in the characterization of brain tumors and shed some important light on the potential role of MRS in the differentiation of various tumor types. It concluded that MRS was accurate in differentiating high- and low-grade tumors. Specifically, based on receiver operating characteristic (ROC) curve analysis of multiple studies examining sensitivity and specificity of proton MRS for differentiating high- from low-grade tumors, the study found excellent values for this purpose in the AUC in multiple studies. In studies by Devos and Lukas and colleagues, AUC values of 94% and 96% in studies using long and short TE, respectively, were obtained. In Hollingworth’s meta-analysis, two studies also showed excellent ability of proton MRS to differentiate between high- and low-grade gliomas; specifically, Herminghaus and coworkers showed in their study of 90 patients that the sensitivity and specificity of proton MRS for the differentiation of high-grade from low-grade tumors were 95% and 93%, respectively. In addition, Astrakas and associates demonstrated in their cohort of 66 patients that by using a combination of MRS parameters (namely Cho/Cr and Lac/Cr; threshold value = 1.8), an AUC value of 96% was obtained for the sensitivity and a specificity of 88% for differentiation of high-grade from low-grade tumors.
Astrocytomas typically demonstrate reduced NAA levels, moderately reduced Cr levels, and elevated Cho levels compared to normal brain parenchyma ( Fig. 17-3 and Table 17-1 ). These absolute reductions result in abnormally low NAA/Cr ratios and elevated Cho/Cr ratios (see Table 17-1 ). NAA levels in astrocytomas are reduced to 40% to 70% of the levels seen in normal brain parenchyma. Furthermore, Cho has been reported to be substantially elevated in more malignant astrocytomas, and this may be secondary to increased cellularity and cell membrane synthesis. There is evidence to suggest that the elevation of Cho is proportional to the degree of tumor malignancy. However, highly malignant primary brain tumors with extensive necrosis may actually demonstrate decreased levels of Cho. Elevated Cho levels are seen more consistently in anaplastic astrocytomas (which do not demonstrate histologic evidence of necrosis) than in glioblastoma multiforme (GBM), and ependymomas display higher Cho/Cr ratios than those noted for astrocytomas in general.
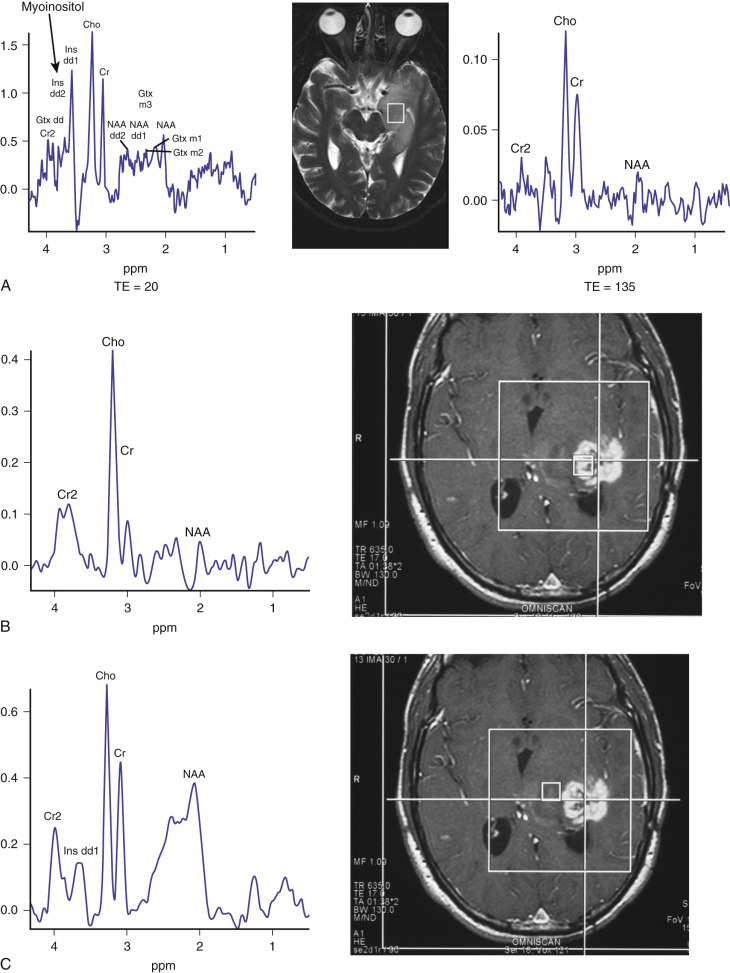
TE = 135 msec | TE = 30 msec | ||
---|---|---|---|
(Cho/Cr) | (Cho/Cr) | (MI/Cr) | |
Normal (n = 20) | 1.08 ± 0.19 | 0.91 ± 0.15 | 0.42 ± 0.16 |
Grade 1 (n = 5) * | 2.25 ± 0.44 | 1.57 ± 0.26 | 1.95 ± 0.74 |
Grade 2 (n = 5) † | 1.66 ± 0.28 | 1.28 ± 0.63 | 1.02 ± 0.13 |
Grade 3 (n = 5) | 2.72 ± 0.49 | 2.06 ± 0.36 | 0.49 ± 0.21 |
Grade 4 (n = 10) | 3.23 ± 1.06 | 2.15 ± 0.66 | 0.33 ± 0.17 |
* WHO grade 1 lesions were biopsy-confirmed pilocytic astrocytomas.
Lac levels may be elevated in some astrocytomas as well. However, a higher incidence of Lac in more aggressive or higher-grade astrocytomas is controversial because Lac may also be present in benign pilocytic astrocytomas. It is thought that Lac may be present across the entire spectrum of grades of astrocytomas because Lac may arise not only from hypoxia developing within the tumor itself as a result of disruption of normal vascular networks but also from necrosis or cysts within the tumor. Lac levels may be elevated in all cysts regardless of etiology.
The presence of Lac has been attributed to (1) the extent of anaerobic glycolysis, (2) the efficiency of electron transport, and (3) the rate of washout from tumor tissue. In highly vascular tumors, Lac may be rapidly removed from the tumor as a result of increased blood flow; therefore, even in high-grade vascular tumors, Lac may not be present in the MR spectra.
The value of Lac identification following radiation therapy or surgery is controversial, but the Lac concentration may be proportional to the original tumor size. Lac has been reported following radiation therapy and surgery, including stereotactic biopsy, although postsurgical porencephaly may lead to artifactual increases in Lac levels because cerebrospinal fluid (CSF) is known to be rich in Lac.
The presence of elevated lipid levels has also been used to differentiate low-grade from high-grade neoplasms. Some studies suggest that mobile lipids tend to be present in higher-grade neoplasms, with highest levels noted in GB; however, although high levels of mobile lipids may be specific for anaplastic astrocytoma or GB, their absence in MR spectra does not exclude the possibility of such high histologic grades. Lipids may originate from tumor cells within high-grade astrocytomas, macrophages along the tumor periphery, or areas of necrosis. Lipids may be present as a result of microscopic cellular necrosis, which may not be apparent on conventional MRIs.
There are inherent limitations of histopathologic grading of gliomas that make it a somewhat imperfect gold standard for tumor assessment. For example, there is the intrinsic sampling error associated with current stereotactic biopsy, which is frequently based on enhancing components of tumor, when HGGs are known to infiltrate nonenhancing parenchyma following the vascular channels of white matter tracts. Furthermore, in adults the biological behavior of low-grade astrocytomas with identical histologic grades is heterogeneous, with more than half of low-grade tumors eventually recurring as or evolving into more aggressive tumors. Some investigators believe that differences in MRS ratios of various metabolites, like differences in glucose metabolism in positron emission tomography (PET), may be of prognostic significance even if they are not of diagnostic value in such cases of low-grade neoplasms. Physiologic MRI, such as proton MRS, not only allows whole lesion evaluation but also allows for evaluation of metabolic abnormalities beyond the tumor margins.
Defining and Delineating Metabolically Different Tumor Regions
A major potential role for proton MRSI is in treatment planning and monitoring of tumor response. Clinical oncologists face a challenge when attempting to implement a treatment protocol that not only will effectively treat the patient’s central nervous system (CNS) tumor to improve survivability but also maintains the patient’s neurologic functions and avoids major treatment-associated morbidities. The capability of MRI to obtain anatomic images in any orientation and to vary between T1- and T2-weighted contrasts has greatly aided in identifying regions containing tumor and in the planning and monitoring of surgical and radiotherapeutic treatments. Furthermore, the use of intravenous Gd-containing contrast agents along with perfusion and water diffusion MRI techniques allow more accurate differentiation of tumor from edematous regions.
However, confirming the presence and extent of malignant disease with conventional MR techniques is still problematic. This is especially true for highly diffuse primary brain tumors. For instance, not all visible MR Gd contrast enhancement may correspond to the presence of tumor. Other biological processes besides the presence of a tumor can cause breakdown of the blood-brain barrier, which leads to contrast enhancement. Tumefactive inflammatory processes and treatment-induced necrosis are known to cause breakdown in the blood-brain barrier, leading to an MR appearance suggestive of tumor process. In addition, absence of Gd contrast enhancement does not always indicate that tumor is not present. WHO grade 2 gliomas commonly do not exhibit Gd contrast enhancement, and furthermore the heterogeneity of HGGs suggests that some regions of the tumor may enhance while other regions of the same tumor do not. Therefore treatments based solely on Gd contrast–enhanced T1-weighted MRIs to delineate the extent of the tumor may not target the full extent of the tumor and/or the most active regions of the tumor. Similar ambiguities with T2-weighted and FLAIR MR tumor imaging have also been found.
Accurate diagnosis and delineation of tumor extent is critical to the clinical management of patients with intracranial tumors. Both surgical and radiotherapy tumor treatment plans are based on the conventional MRI techniques described previously and have been shown to be inadequate by themselves to accurately diagnose and delineate tumor extent. Depending on the tumor type, these MR techniques can only diagnose intracranial lesions with a 30% to 90% success rate. Biopsy is still considered the gold standard for determining cancer type and degree of malignancy. Once the lesion is diagnosed as a malignant brain tumor, planning of the surgical and radiotherapy treatments is based solely on the anatomic abnormalities observed on the conventional MRI studies. These conventional MRI studies, as indicated earlier, do not always provide an accurate picture of the extent or location of the tumor. This is especially true in determining the zone(s) of tumor infiltration with low to moderate rates of proliferation, regions of nonmigrating actively proliferating tumor, and regions of quiescent pseudopalisading migrating hypoxic tumor cells adjacent to necrotic regions. Usually, large uniform surgical and radiotherapy margins between 1 and 4 cm are treated to account for these regions. These margins may underestimate the extent of tumor infiltration, treat areas devoid of tumor (which increases the risk for normal tissue toxicity events), and/or undertreat tumor cells that are not actively proliferating in the planned treatment volumes.
To obtain the most benefit from the newer neurosurgical and radiotherapy treatment approaches, such as computer-guided stereotactic neurosurgical techniques and the use of intensity-modulated and conformal radiotherapy treatment techniques, it is important that regions identified for special attention be defined accurately. Areas of active tumor proliferation need to be identified from areas suspicious for tumor extension, which are less proliferative but more infiltrative from areas that contain quiescent pseudopalisading migratory tumor cells around regions of necrosis. Noninvasive techniques that can provide information to improve our ability to characterize the genetic and molecular differences in the tumor populations present in a lesion and delineate the spatial extent of each population need to be utilized. This information, especially in HGG patients, may improve our ability to treat these lesions more accurately with the techniques now available and aid in the development of more targeted therapies to obtain better patient outcomes.
In 2001, Dowling et al. took an important step in establishing MRS as a potential technique to aid in the mapping of tumor regions. Using a three-dimensional (3D) MRS technique, they evaluated the 3D MR spectra obtained from 28 brain tumor patients and correlated the histologic findings from specific sites to the corresponding MR spectral voxels. Their results showed the following:
- (1)
If the level of NAA is normal, the tissue within that voxel is normal. Conversely, abnormal levels of NAA correlate with abnormal tissue regardless of the underlying histologic findings ( Figs. 17-4 and 17-5 ).
FIG 17-4
Proton MRSI of uninvolved brain region adjacent to low-grade lesion. Note level of NAA in abnormal FLAIR tumor region vs. uninvolved tumor region shown in square.
FIG 17-5
Infiltrative grade 4 glioma (A,B) . Note level of Cho relative to Cr and NAA in enhancing and nonenhancing brain regions. Both spectra are indicative of tumor being present.
- (2)
When a lesion contains a Cho peak area intensity greater than the peak area intensity of NAA and larger than the normal peak area intensity of Cho, tumor was always present (see Figs. 17-5 and 17-6 ).
FIG 17-6
SPGR (spoiled gradient recalled acquisition in steady state) image and proton MRS spectral location of a patient with an oligoastrocytoma. A, MRI location and the MRS spectrum for a biopsy that yielded 75% tumor. Note the elevated Cho resonance and absence of NAA in this voxel. B, MRI location and MRS spectrum for a biopsy that correlated with the pathologic finding of necrosis, astrogliosis, and white matter. Note the low levels of Cho, Cr, and NAA resonances in this voxel region. Although the MRI intensity is similar to the region in A, significant differences in both the spectra and pathologic findings were found in these regions.
(From Dowling C, et al: Preoperative proton MR spectroscopic imaging of brain tumors: Correlation with histopathologic analysis of resection specimens. AJNR Am J Neuroradiol 22:604–612, 2001.)
- (3)
The peak area intensity of Cho correlated with percentage of tumor in the sampled voxel.
- (4)
Undetectable to near-normal levels of NAA and Cho are seen in areas of gliosis and necrosis ( Fig. 17-6 ).
- (5)
Image areas with similar appearance and enhancement may show different spectral patterns and histologic characteristics (see Fig. 17-6 ).
These observations suggest that 3D MRSI could be a potentially important method that can be used to define more accurately tumor margins, identify areas with the highest proliferative fractions, and identify posttherapy sites of tumor recurrence. For example, Pirzkall et al. studied whether the metabolic information provided by MRSI-defined volumes could improve the accuracy of target volumes for radiotherapy treatment planning compared to target volumes defined by MRI. Using elevated Cho and decreased NAA as criteria, Pirzkall et al. compared the MRSI-defined volumes to that defined by the Gd-enhanced T1- and T2-weighted MRI studies. They found that the T2 studies estimated, as judged by the volume of the hyperintense T2 region, the region at risk of containing microscopic disease as being as much as 50% greater than by MRSI. On the other hand, the Gd-enhanced T1 MRI studies suggested a smaller tumor volume and a different location for highly active disease compared to the MRSI study ( Fig. 17-7 ). They concluded that “the use of MRSI to define target volumes for RT treatment planning would increase, and change the location of the volume receiving a boost dose as well as reduce the volume receiving a standard dose.” This study suggests that inclusion of MRSI data in the treatment planning process could potentially improve tumor control while reducing normal tissue radiation complications. This was clearly shown to have occurred in an external beam radiation therapy study of 26 patients with grade 4 gliomas in a study conducted by Chan et al. Patients with MRSI abnormalities (i.e., elevated Cho/NAA levels) outside of the MRI-defined target volume had decreased median survival times relative to those with MRSI abnormalities inside the MRI-defined target volumes (10.4 vs. 15.7 months).
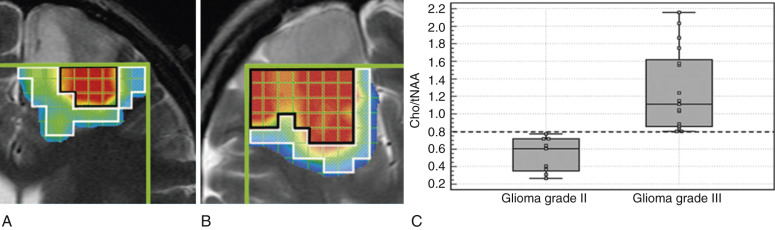
Applying a multiparametric MR scheme using information from H1-MRSI, PWI, DWI, and conventional MRI, Di Constanzo et al. found that the combination of these techniques provided useful information that could be used to further define and improve the delineation and extent of tumor processes. In particular, this multiparametric study characterized more fully some of the more extreme spatial heterogeneities of tumor and could identify patterns for just tumor and edema alone, tumor and edema patterns in perienhancing regions of interest (ROIs) with abnormal signals on conventional MRI, and a tumor infiltrated pattern in apparently normal-appearing perienhancing ROIs. More importantly, this study suggested that changes in the MR parameters NAA, Cho, ADC, and rCBV could accurately classify the different perienhancing ROI patterns and be used reliably for guiding stereotactic biopsies, surgical resections, and radiation treatments of these regions.
MRS Spectral Profile of Proliferative, Hypoxic/Pseudopalisading, and Infiltrating Glioma Populations
One of the major factors that contributes to the poor therapy outcome in HGG patients is the subjective grouping of all HGGs by only their histologic characteristics, without considering the differences in molecular and genetic characters of different tumor populations within the observed HGG—namely, the main proliferating and stationary tumor body, the infiltrative population of HGG (which has a lower proliferation rate), and the quiescent pseudopalisading migratory tumor population adjacent to necrotic regions of the lesion. The molecular and/or genetic characteristics of these populations can affect different proliferative and/or infiltrative signaling pathways that are not normally considered when designing treatment plans. For example, with radiotherapy treatment of HGG, concomitant temozolamide chemotherapy is delivered; the reason for this is the mechanism of action of this drug. Temozolamide is a DNA alkylating agent that induces nicks in DNA, and if not repaired, daughter cells can inhibit replication, leading to increased apoptosis and increased radiation sensitivity. Inhibition of cellular replication occurs in the G2-M phase of the cell cycle, the most radiosensitive phase of the reproductive cycle. Thus the major effect of temozolamide is on tumor cells in the most radioresponsive portion of the cell cycle. However, the level of response to temozolamide has been shown to be dependent on the genetic character of the HGG. Deletion on chromosome 10 (PTEN) of the phosphatase and tensin homolog leads to increased sensitivity to temozolamide. PTEN is a lipid phosphatase that plays a basic role in attenuating the phosphatidylinositol 3-kinase (P13K)/Akt-1 signaling pathway; hence loss of PTEN can affect major oncogenic events during glioma genesis. Loss of PTEN occurs in about 36% of patients diagnosed with grade 4 gliomas; thus only about a third of the patients diagnosed with HGG will benefit from temozolamide treatment.
However, what about the two other tumor populations present in the HGGs in patients who respond to temozolamide—namely, the pseudopalisading migratory cells adjacent to necrotic regions and diffusively infiltrating tumor cells? Both of these tumor cell types should be refractory to temozolamide, because neither proliferate rapidly.
Around areas of necrosis in HGG, the tumor cells show psuedopalisading. Data suggest that the pseudopalisading zone is the result of tumor cells migrating away from the necrotic region, and that necrosis selects for tumor cells that are more aggressive and more resistant to apoptosis-inducing therapeutic regimens. Similarly, data indicate that glioma cells that migrate out of the main tumor body have a decreased proliferative capacity and decreased susceptibility to apoptosis. These two populations may be the tumor cells responsible for regrowth and recurrence of HGG. Thus more information should be obtained that characterizes molecular signaling pathways activated in these populations, which will allow for more effective therapies to be developed. In the case of the migratory HGG cells, evidence indicates that treatment with inhibitors of migration does not affect apoptosis of migration-restricted/stationary glioma cells but can sensitize migrating glioma cells to cytotoxic agents.
Proliferative Glioma Population: Cho/NAA Ratio, ADC, and rCBV Parameters.
At present, the major role of MRSI in the surgical treatment planning for HGG is in guiding the neurosurgeon to regions that show high metabolic activity on biopsy (regions with elevated Cho levels and low NAA levels relative to normal brain tissue). In a study conducted by Gupta and colleagues, 18 glioma patients were preoperatively examined with H1-MRSI and DWI along with conventional MR studies. This study was conducted to correlate quantitative presurgical H1-MRSI and DWI results with quantitative histopathologic characteristics of resected tumor tissue. The primary hypotheses to be tested were: “(1) glioma choline correlates with cell density, (2) glioma apparent water diffusion coefficient (ADC) correlates inversely with cell density, and (3) glioma choline signal correlates with cell proliferative index” as measured by MIB-1 immunostaining. The tumor-to–contralateral normalized Cho signal ratio (nCho = Cho tumor voxel /Cho contralateral normal volume ) and the ADC from resected tumor regions were determined from the preoperative MRI studies. The results showed that cell density correlated linearly with nCho but inversely with ADC values. No correlation was found between nCho and cell proliferative capacity.
However, in a similar study conducted by McKnight et al., using a normalized Cho/NAA (CNI) and Cho/Cr (CCrI) index instead of nCho index, they found that the normalized Cho/NAA but not the Cho/Cr ratios correlated significantly with the proliferation index of the tumors as determined by MIB-1 immunostaining ( P < 0.001) and cell density measurements ( P < 0.001) of the biopsy-obtained voxel sample. This finding suggests that voxels containing elevated normalized Cho/NAA ratios have the highest probability of being a region that has “high cell density, high proliferative fraction, and/or high ratio of cell proliferation to cell death.”
As discussed previously, for surgical, chemotherapeutic, and radiation therapy clinical patient treatment decisions it is important to be able to delineate and identify, as confidently as possible, the various heterogeneous regions of the tumor so that the correct treatment plans can be applied to these regions. Thus the more noninvasive parameters or changes in the values of the parameters one can use to identify the tumor ROIs, the more accurate and more reliable the tumor map will be, which can be used to devise the most effective treatment paradigm for the patient. In the case of proliferative tumor regions, addition of the rCBV maps obtained from PWI studies can be used to further identify and delineate the major proliferating regions of the tumor. Advanced MRI techniques have been developed that allow noninvasive study of tumor vascularity. Dynamic susceptibility-weighted contrast-enhanced perfusion MRI, used widely in clinical practice, relies on the T2* signal intensity change that occurs when the contrast agent passes through the tissues. This change in signal intensity allows calculation of the rCBV, and this parameter has been correlated with tumor vascularity. Studies have shown that gliomas that have higher rCBV also have higher mitotic activity, as judged from the histopathologic determination of the number of mitotic cells found in tissue sections from stereotactic biopsies obtained from the perfusion MRI study. More recently, Price et al. has shown that the rCBV in HGGs linearly correlated with cellular proliferation, as judged by MIB-1 immunostaining and histopathologic analysis of resected tumor section analyzed from preoperative PWI; r = 0.66, P < 001). Thus, using the correct thresholds for the Cho/NAA ratios, ADC, and rCBV for proliferating tumor regions, and coregistering this information with the anatomic MRIs can be used to improve the delineation and more accurately assess tumor regions having high proliferative capacity.
Hypoxic/Pseudopalisading Tumor Population: MRS Metabolic Profile and the Role of PET Imaging.
Two other cell populations within the tumor that can lead to recurrence and/or resistance to therapy also need to be evaluated: (1) the quiescent pseudopalisading migratory hypoxic tumor population adjacent to necrotic regions and (2) the active infiltrating but low mitotically active tumor population migrating from the main tumor body. If neither of these populations are either resected or targeted for further treatment, they will lead to repopulation/recurrence and spread into other areas of the brain. In the case of the hypoxic/pseudopalisading population, this population is normally quiescent and if not surgically resected will be more resistant to standard radiotherapy treatments normally applied following surgery; this will lead to repopulation and spread of the tumor. In addition to their intrinsic resistance to radiation damage, this hypoxic tumor population is often refractory to the cytotoxic actions of conventional chemotherapeutic agents. Thus it is important to try to identify the location of this population for the design of augmented surgical, radiotherapy, and chemotherapy treatment plans.
MRSI and conventional MRI, in conjunction with positron emission tomography (PET) of [F-18] fluoromisonidazole uptake (F18-FMISO) may be of use to map out the regions containing the hypoxic/pseudopalisading tumor cells. F18-FMISO is a fluoronitroimadazole compound that was one of the first PET agents used for the pretherapy detection of hypoxic tumor populations in patients with tumors outside the CNS. With the advent of PET-MR clinical scanners, PET studies can be readily registered into the MRI and MRSI studies to produce anatomic maps combined with functional maps showing the distribution of proliferating and quiescent tumor populations in hypoxic regions. F18-FMISO PET can be used to image tumor hypoxia, because tumor cells show increased F18-FMISO uptake, and once taken up, it is exclusively trapped in hypoxic tumor cells because of the reduced cellular oxygen environment of these cells. F18-FMISO is cleared in normoxic tumor cells.
The presence of hypoxia appears to be a common feature of HGGs that accumulate F18-FMISO. The hypoxic regions found in HGG have been shown to be associated with the regions adjacent to areas of necrosis where the hypoxic pseudopalisading cells reside. Recently Bruehlmeier et al. found that uptake and retention of F18-FMISO was consistently found in the periphery of the lesion adjacent to necrotic regions of the HGG.
MRSI can add support to the F18-FMISO PET findings because it has been shown that regions showing low Cho and NAA levels relative to normal brain normally indicate necrosis, astrogliosis, or regions with macrophage infiltration. However, these regions that appear to be necrotic may also contain quiescent pseudopalisading migratory tumor cells adjacent to necrotic centers, especially if they show spectra (obtained at TE = 30 milliseconds) that have elevated Cho/NAA and Cho/Cr ratios, presence of lipid/Lac resonances, but overall lower levels of metabolites relative to normal tissue ( Fig. 17-8 ). This spectral profile in fact may represent the most aggressive tumor population within HGGs and the major population responsible for the resistance and repopulation of tumors.
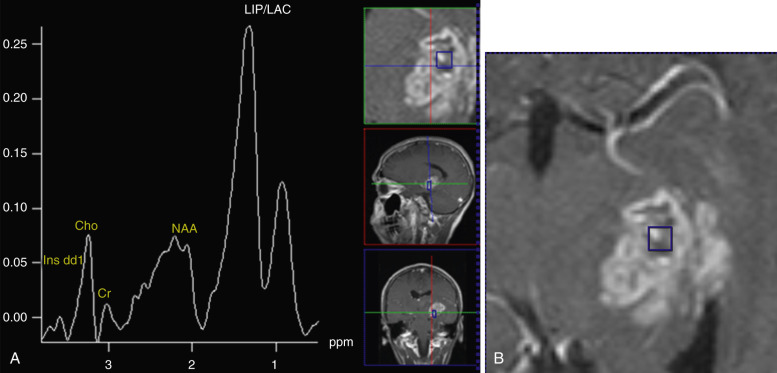
In a study conducted by Martin et al. to determine the efficacy of MRSI in guiding biopsy, they found that in 17 out of 21 patients who had histologically confirmed biopsy tumor samples, the Cho levels were elevated relative to normal levels. However, in four patients with low Cho levels similar to those observed in necrotic regions, histologic evaluation of the biopsy sampled showed tumor. The representative MRSI spectra of one of these patients ( Fig. 17-9 ) shows relatively elevated Cho/Cr and Cho/NAA ratios and an elevated lipid/Lac resonance, suggestive of a necrotic region with actively proliferating tumor cells.
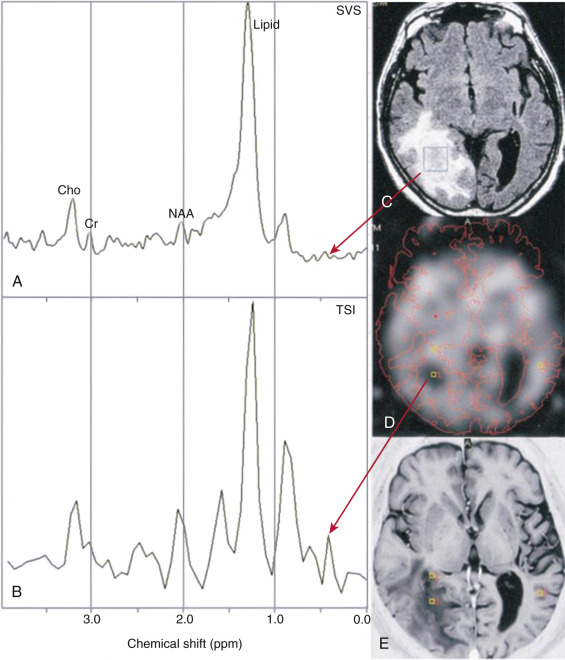
Infiltrative Glioma Population: Cho/Cr and Cho/NAA Ratio Profiles, rCBV, and Fractional Anisotropy.
When targeting tumor biopsies or defining tumor treatment volumes, the infiltrating tumor population must be taken into account. To determine the usefulness of MRSI in assessing the degree of tumor infiltration, Ganslandt et al. performed MRSI studies on 7 patients with untreated grade 2 and 3 gliomas. The MRSI data sets were fused with the 3D MRI data sets and integrated into a frameless stereotactic system for image-guided interactive neuronavigation surgery. Tissue samples were obtained from three regions based on the Cho/NAA ratios found in the MRSI study: (1) normal-appearing brain region, (2) zone between spectroscopically pathologic and suspicious for infiltrating tumor volumes, and (3) maximum spectroscopically pathologic tumor volumes. The implementation of the MRSI data set into the frameless stereotactic system was successful in all cases, and stereotactic biopsies were obtained from the MRSI-defined regions. In this study, a relationship between the tumor cell density and Cho/NAA metabolic changes were found (60%-100% in the maximum pathologic areas to 5%-15% in the border zones suspicious for infiltrating tumor). Another important finding of this study was that the tumor areas defined by the metabolic maps, which were confirmed histopathologically, exceeded the T2-weighted abnormal signal change in all cases by 6% to 32%. However, in four patients, biopsies obtained with normal Cho/NAA ratios showed the presence of tumor infiltration. It was suggested that the false-negative finding in these patients was due to the low resolution of MRSI with respect to the glioma borders. Another explanation could be that the Cho/NAA ratio reflects primarily the proliferative capacity of the tumor cells and not necessarily the migratory capacity. Thus in infiltrative tumor zones, Cho/NAA levels may be normal or only slightly elevated because tumor cells are not dividing and/or displacing or destroying normal neural cells. Highly infiltrative cells normally only proliferate at vascular branch points, therefore in infiltrative white matter tumor regions the Cho/NAA ratios may not be elevated. However, the Cho/Cr ratios may be elevated. This is shown by Wright to represent an infiltrative spectral tumor pattern.
The infiltrating tumor pattern of gliomas is closely associated with the expression of matrix metalloproteinase 2 (MMP-2). Invasion of glioma cells involves the attachment of invading cells to the extracellular matrix (ECM), disruption of the ECM components, and subsequent penetration of the invading cell into adjacent brain structures. This is accomplished in part by the secretion of MMPs by the invading glioma cell to break down the ECM barrier, which impedes the infiltrating tumor cells from extending. Zhang et al. found a significant correlation between the Cho/NAA and Cho/Cr ratios and MMP-2 expression; it appears that the higher the ratios, the more invasive/infiltrative the tumor. This is in agreement with the image-guided MRSI brain tumor biopsy studies of Croteau et al. Histologic analyses of tissue samples obtained from the MRSI-guided biopsy sites found that using contralateral Cr and Cho levels for normalization or ipsilateral NAA appeared to correlate with the degree of tumor infiltration. They found that the degree of tumor infiltration increased with increasing Cho/NAA ratio and Cho/Cr ratios (minimal level of tumor infiltration had Cho/NAA = 1.09 ± 0.12 and Cho/nCr = 1.62 ± 0.09; maximum level of infiltration had Cho/NAA = 2.01 ± 0.18 and Cho/nCr = 1.98 ± 0.18).
Using the Cho/Cr and Cho/NAA threshold ratios defined in the Croteau study for minimal level of tumor infiltration may be useful in delineating the more metabolic and proliferative tumor populations ( Fig. 17-10 ) from the less proliferative infiltrating tumor ones ( Figs. 17-11 and 17-12 ). Figure 17-11 shows a tumor that appears to be infiltrating into areas beyond the Gd T1-weighted enhancing regions of tumor. The Cho/Cr peak area ratios in these areas are 1.5 or greater. The region outside the enhancing area also shows Cho/NAA peak area ratios of 1.0 or less. This suggests that in areas outside the Gd-enhancing or T2-weighted hyperintense areas, in which Cho/Cr ratios greater than 1.5 and Cho/NAA levels of less than 1.0 occur (see Fig. 17-12 ), there may be regions containing a mixture of normal cells and infiltrating tumor cells with lower rates of proliferation. In the area of enhancement or extending just beyond this area, where the MRSI Cho/Cr ratios are greater than 1.5 and the Cho/NAA ratios are greater than 1.0, are areas of active tumor proliferation and infiltration (see Fig. 17-11 ). The spectral pattern observed for the infiltrative tumor process is consistent with the infiltrative tumor spectral pattern found by Wright et al. ( Fig. 17-13 ).
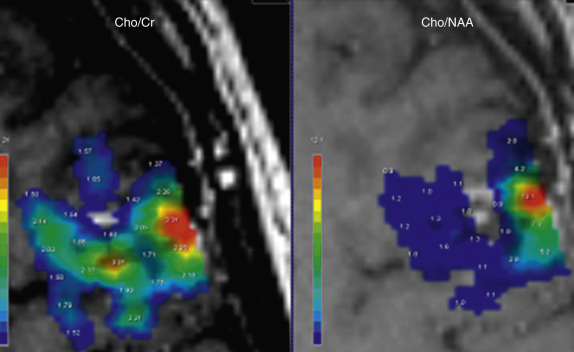
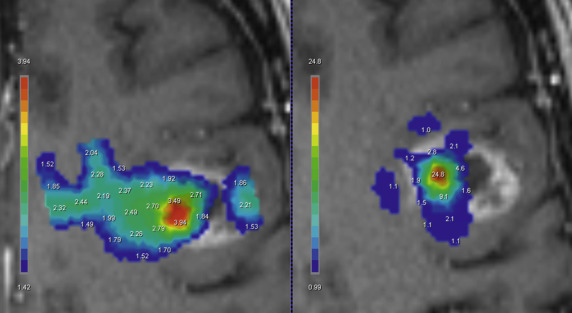
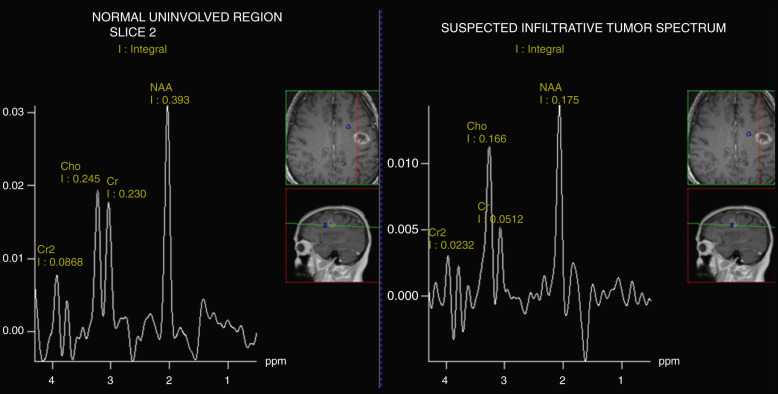
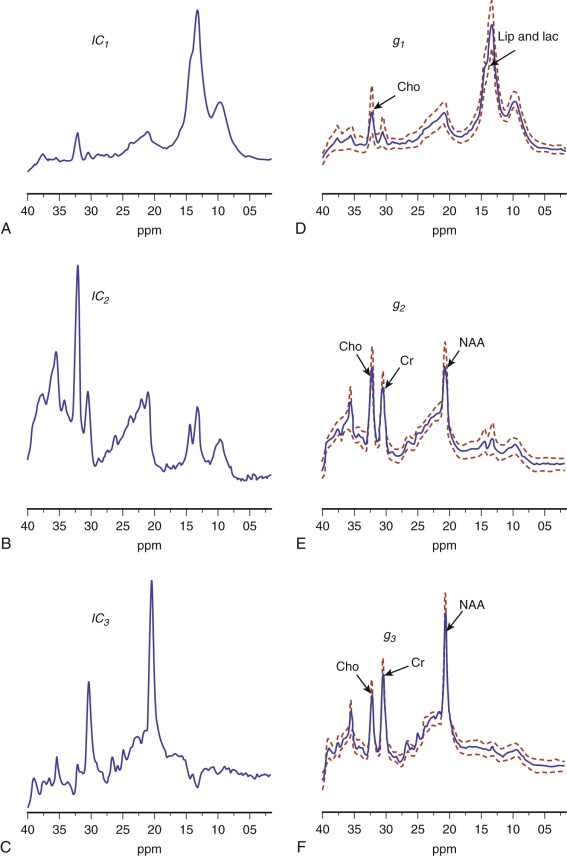
Cho/NAA ratios may be low (<1.0) in areas of tumor infiltration, because the normal neuronal cells are not being eliminated in these regions by actively proliferating tumor cells, as would be expected in regions in and peripheral to the Gd-enhancing area where Cho/NAA ratios are greater than 1.0. Figure 17-10 shows an HGG that appears to be well circumscribed. The Cho/Cr ratio is greater than 1.5 only in or adjacent to the enhancing region where the Cho/NAA peak area ratio is greater than 1.0. It does not appear to extend beyond this region as defined by the Cho/Cr peak area ratio of 1.5 or greater for tumor threshold (namely, where the Cho/Cr is > 1.5, it is highly probable tumor is present in this voxel). This observed Cho/Cr and Cho/NAA ratio pattern may suggest that the tumor is expanding via a more proliferative rather than an infiltrative pathway; this may be due to the more cortical location of this lesion, which predominantly affects white matter.
Di Constanza et al. used an MR multiparametric approach to assess the extent and malignancy of cerebral gliomas. Thirty-one patients (21 high grade and 10 low grade) with gliomas underwent conventional MRI, H1-MRSI, DWI, and PWI studies prior to surgery and histologic examination of resected tissue. ROIs with high Cho (>1.3; Cho volume on tumor side/Cho contralateral normal volume ) and or abnormal Cho/NAA greater than 1.0 (normalized to ratio found in contralateral volume) were considered tumor or infiltrated tumor on the basis of previous neuropathologic correlation studies.
From this multiparametric study of high-grade lesions, three tumor regions could be delineated: (1) Gd-enhancing tumor region (margin and mass), (2) perienhancing abnormal region containing tumor and edema, and (3) normal-appearing perienhancing region containing infiltrated tumor and normal brain parenchyma. The high-grade lesions containing the bulk tumor mass and enhancing margins (1) showed very high mean Cho/NAA ratios greater than 4.0 and Cho/Cr greater than 2.0 (normalized relative to contralateral normal region), high mean ADC values (1.24 ± 0.31 × 10 −3 mm 2 /sec in bulk tumor vs. 0.77 ± 0.05 × 10 −3 mm 2 /sec in normal brain region), and mean elevated rCBV of 3.70 in tumor vs. 0.98 in normal tissue. In the perienhancing abnormal-appearing region containing tumor and edema (2), the normalized mean Cho/NAA was found to be 3.3 and Cho/Cr was 1.76, the mean tumor ADC value was 0.92 ± 0.17 × 10 −3 mm 2 /sec, and mean tumor rCBV was 1.68 ± 0.41. In the normal-appearing perienhancing region (3), the normalized mean infiltrated tumor Cho/NAA ratio was 1.55 and Cho/Cr ratio was 1.28, the mean ADC of this region was 0.86 ± 0.08 × 10 −3 mm 2 /sec, and the mean rCBV of this region was 1.28 ± 0.16.
The primary change in the ratios of Cho/NAA in the normal-appearing infiltrated tumor region of a high-grade lesion is due to a change in the Cho levels and not to changes in the level of NAA. The mean normalized NAA levels in infiltrated tumor regions were 0.94 ± 0.23 vs. 0.96 ± 0.14 for the levels found in the normal contralateral side. In the bulk tumor mass, the mean NAA level was 0.31 ± 0.11, and in the perienhancing abnormal region (2) the mean NAA level was 0.58 ± 0.22. Thus in both regions 1 and 2 (as per above), the increased changes observed in the metabolite ratios appear to be due to decreased levels of NAA and increased Cho. This suggests higher tumor proliferative capacities in these regions as measured by increased Cho levels and loss of NAA levels. NAA is a neuronal marker, so the loss of NAA suggests either loss of neuronal activity or destruction due to tumor proliferation and invasion into these regions. However, in region 3 the almost normal level of NAA suggests that changes observed are due primarily to infiltrating tumor cells that are not proliferating rapidly. This interpretation is consistent with the changes in rCBV observed in tumor regions (2-3) of this study. Price et al. showed that high-grade brain lesions that have increased mitotic activity also have higher rCBV values. These investigators showed that rCBV correlated significantly with MIB-1 labeling index and not with tumor cell packing, which suggested that increased tumor vascularity as measured by rCBV was more dependent on cellular proliferation than just the number of cells present.
Low-grade lesions demonstrated the same trend with respect to Cho/NAA, Cho/Cr, ADC, and rCBV values in bulk tumor versus infiltrated tumor regions. The bulk of the tumor showed much higher values for all parameters compared to the normal-appearing adjacent infiltrated tumor regions, but in both the bulk tumor and the infiltrated tumor regions, values were found to be higher than in the contralateral normal brain regions.
Tumor infiltration into white matter can induce widening of white matter fiber bundles, which affects the directionality of the motion of water molecules within the white matter fiber bundles. Changes in the directionality of water molecules within white matter tracts can be measured with DTI. By employing at least six diffusion gradient directions, compared to three gradient directions, DWI studies enable not only the macroscopic detection and imaging of the highly anisotropic water diffusion found in white matter bundles but can also detect changes in the anisotropic motion of water (i.e., directionality) within white matter due to tumor invasion, which cause disruption of white matter bundles. A DTI study generates a diffusion tensor D for each image voxel, and from each D voxel value, the magnitude and directionality of water diffusion can be calculated. The magnitude is known as the mean diffusivity (MD) and is mathematically equivalent to the ADC obtained from a conventional DWI study. The directionality is known as fractional anisotropy (FA).
In a 2006 publication, Stadlbauer et al. used DTI to retrospectively correlate changes in FA and MD with the degree of tumor cell infiltration determined histologically. Histopathologic findings of 77 MRI-guided stereotactic biopsies in 20 glioma patients were correlated with the FA and MD values found at the biopsy sites. FA was found to be inversely correlated to percent tumor infiltration and was found to be better than MD for assessment and delineation of tumor infiltration. For low tumor cell numbers as shown in Figure 17-14 , the logarithmic regression lines showed a strong decrease in FA (see Fig. 17-14A ) and an increase in MD (see Fig. 17-14B ) followed by an asymptotic plateauing trend for high tumor cell number. From these findings, the investigators hypothesized that their findings “reflect the infiltrative invasion mechanism of gliomas”—namely, initial enlargement of the extracellular space as the tumor invades, leading to a logarithmic decrease in FA and a logarithmic increase in MD but preserving the normal microstructure of the white matter fiber bundles in the border zone. This hypothesis is consistent with the data of Di Constanza et al. in which the NAA levels remained relatively unchanged while the Cho levels increased by almost 50% in suspected tumor-infiltrated normal-appearing ipsilateral white matter. This lack of change in NAA reflects only the initial phase of tumor infiltration into these regions, which induces only mild disruption of white matter bundles. As more tumor cells infiltrate and proliferate, there is a decrease in the extracellular space (e.g., edema) and a decrease in directionality of diffusion due to tumor-induced derangement and destruction of white matter microstructures, which leads to decreases in NAA along with increased Cho levels.
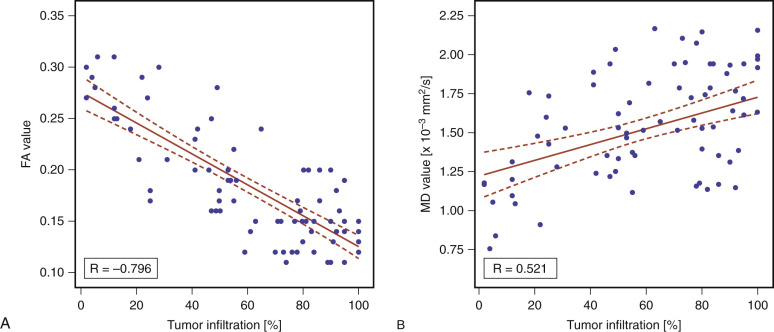
A number of investigators have proposed the combined use of proton MRSI and DTI to map out the infiltrative regions of gliomas. Wright et al. performed independent component analysis of MRSI data from human gliomas to segment tissues into tumor core, tumor infiltration, and normal brain. DTI analysis was then used to confirm the correctness of the MRSI segmentation pattern (see Fig. 17-13 ). They found that the MD and FA data found for these regions were consistent with previous studies that compared anomalies in isotropic and anisotropic diffusion images to determine regions of potential glioma infiltration ( Table 17-2 ). They showed that coefficients of independent components from their analysis could then be used to create colored images overlaid onto conventional anatomic images to visualize regions of infiltrative tumor growth.
FA | MD | |||||
---|---|---|---|---|---|---|
g 1 | g 2 | g 3 | g 1 | g 2 | g 3 | |
Median | 0.128 | 0.233 | 0.240 | 1.23 (×10 −3 ) | 1.13 (×10 −3 ) | 0.909 (×10 −3 ) |
Significantly different from g 1 (Mann-Whitney U test) | — | Yes | Yes | — | Yes | Yes |
P = 7 × 1048 −48 | P = 3 × 10 −49 | P = 1 × 10 −4 | P = 2 × 10 −30 | |||
Significantly different from g 2 (Mann-Whitney U test) | — | — | No | — | — | Yes |
P = 0.11 | P = 8 × 10 −27 |
MRSI and DTI both provide markers that represent infiltrative growth, and the two techniques are complementary. DTI can detect abnormalities with high spatial resolution, and MRSI can provide validation of the presence of proliferating and/or infiltrating tumor cells from the metabolic profile. The combination of markers will provide greater certainty in correctly identifying regions of tumor infiltration, which can then be treated appropriately.
Monitoring Tumor Response to Therapy
MRS may be able not only to facilitate diagnosis and accurate classification of de novo brain tumors but also to allow differentiation of recurrent tumor and tumor progression from radiation necrosis, posttreatment effects, and edema. Thus MRS may be useful in evaluating the response to therapy in patients with brain tumors. Radiation necrosis occurs from approximately 6 to 24 months after completion of conventional therapy, is seen more commonly with high-grade astrocytomas, and may be indistinguishable from recurrent tumor by conventional Gd-enhanced MRI. Elevated Lac levels are seen in proton MR spectra of patients who have received 40 Gy or more of radiation to the brain, even when the conventional MRI study does not yet demonstrate any structural abnormality within the resection bed.
One study involving 25 patients with cerebral astrocytomas who received a combination of radiation and chemotherapy demonstrated increased Cho/NAA and Cho/Cr ratios as well as the presence of Lac in recurrent tumors, compared to markedly decreased levels of NAA, Cho, and Cr and the presence of a broad intense signal between 0 and 2.0 ppm in cases of radiation necrosis. This broad signal, between 0 and 2.0 ppm, consists of free fatty acids, Lac, and amino acids. However, because most therapy-induced tissue damage occurs in combination with areas of viable tumor, single-voxel techniques are not optimal for evaluation of these patients, and 3D MRSI is preferable for distinguishing among areas of residual or recurrent tumor, radiation necrosis, and viable normal brain tissue. Sensitivity and specificity of proton MRS for the detection of residual/recurrent tumor in radiated patients in one series were 71% and 100%, respectively, and serial MRS in the same series allowed differentiation of necrosis and tumor progression; progressive decreases in Cho levels and mild increases in NAA levels correlated well with therapy success. Elmogy et al. in a 23-patient study with 2D MRSI found that Cho/NAA ratios were the best discriminators of recurrent/residual tumor from radiation injury. In this study using a cutoff of 1.8 for the Cho/NAA ratios, an accuracy of 96%, a sensitivity of 93%, and specificity of 100% were found. In a 3D MRSI of 28 patients, Zeng et al., using a cutoff of 1.71 for either Cho/Cr or Cho/NAA, resulted in a ROC analysis of 96.2% for accuracy, 94.1% for sensitivity, and 100% for specificity of MRSI in discriminating recurrent/residual tumor from radiation injury.
Tedeschi and coauthors demonstrated that interval changes in Cho levels during long-term (3.5 years) follow-up with MRS allowed differentiation of stable from progressive glioma. A study by Henry et al. suggested that MRS and MR perfusion imaging (namely, rCBV mapping) may be complementary modalities that when combined may be better able to noninvasively differentiate tumor from necrosis, posttreatment effects, or edema in patients with treated gliomas than either modality alone.
Graves et al. examined 18 patients with recurrent malignant gliomas with proton MRSI to characterize the temporal tissue responses in these tumors after receiving high-dose gamma knife radiosurgery treatment. Only six patients with recurring high-grade gliomas responded to the treatment and showed reduction of Cho and an increase in Lac/lipid resonances, typically within 6 months post treatment.
Of the 18 patients treated with radiosurgery, 12 recurred after treatment (recurrence was defined as > 2 cc increase in Gd contrast-enhancement areas). In each case, an elevated Cho/NAA ratio was associated with at least one voxel within the area of recurrence. In 9 of the 12 recurring patients, voxels coinciding with the recurring regions showed increased Cho/NAA ratios relative to normal-appearing voxels prior to increases in contrast enhancement. In the remaining 3 patients, the new areas of contrast enhancement appeared in regions and were characterized by pronounced increases in Cho/NAA ratios.
Other groups have reported similar success in distinguishing radiation necrosis from recurrent tumor with proton MRS, although a few have reported contradictory results; the reasons for differences in results may be due to the fact that Cho levels may be elevated in early radiation-induced lesions because of demyelination and reactive astrocytosis. In addition, Bizzi and coworkers reported that MRS is useful in the surveillance of lymphoma following treatment.
Role of Proton MRSI in Predicting Tumor Prognosis
Long-term prognosis for patients with HGG remains dismal even with advances in both imaging and therapy. In a 2003 survival analysis of 766 GBM patients, only 2% of these patients were found to be still alive after 5 years. Given such a bleak prognosis, there is a need to identify noninvasive biomarkers that can be used to characterize individual lesions and predict outcome. This information can then be used to stratify patients into high- or low-risk categories to determine which patients may benefit from new and/or experimental therapies. Several studies have evaluated the role of proton MRSI in the prediction of survival of GBM patients. In a multiparametric MR study of 56 GBM patients, proton MRSI, conventional MRI, DWI, and PWI were combined to examine whether pretreatment characteristics of the tumors obtained from these studies could be used to predict survival of these patients after treatment. This study showed that shorter survival was associated with the volume of tissue with abnormally high Cho/NAA ratio [CNI > 2; (Cho/NAA) tumor vol /(Cho/NAA) normal contralateral vol ], contrast-enhancing tissue and high rCBV, and tissue with abnormally low ADC values. Higher Lac and level of lipids were also associated with a worse prognostic outcome.
Several studies have also examined the prognostic value of proton MRS in evaluating pediatric brain tumors. In a study of 76 pediatric brain tumors, a low Cho/Cr ratio—less than 1.8 index value (normalized to contralateral Cr peak area)—was found to be a strong predictor of survival. In a different report, a high Cho/NAA ratio in children with recurrent gliomas was associated with decreased survival. In a multiparametric longitudinal MRI study of 34 patients conducted at the National Cancer Institute (Bethesda, Md.), Hipp et al. used proton MRS, conventional MRI, and perfusion MRI data to predict outcome in children with diffuse intrinsic pontine gliomas. They found that at baseline MRI only, increased ratio of Cho/NAA and increased perfusion on dynamic susceptibility contrast (DSC)-MRI predicted shorter survival. When examined at later time points, any volumes with an observed maximum Cho/NAA ratio on MRSI (i.e., >4.5) or increased Cho/NAA on either single- or multivolume MRSI compared to baseline increased perfusion, and the presence of enhancement predicted shorter survival. They concluded that changes in Cho/NAA over time, as determined by either single- or multivolume MRS were “prognostic and can potentially be used as indicators of response or lack of response to treatment.” They also recommended that routine baseline and subsequent imaging for children with diffuse intrinsic pontine gliomas should, at a minimum, incorporate both DSC-MRI and single-voxel spectroscopy.
Differentiation of Neoplastic from Pseudotumoral Process
The differential diagnosis of a brain lesion may vary depending on its solid and necrotic characteristics. For example, if the brain mass encountered on a conventional MR examination appears more necrotic in nature, the differential diagnosis would favor that of either an aggressive malignant brain tumor (e.g., metastatic brain lesion or GB) or nonneoplastic pseudotumoral brain lesion that mimics a brain tumor (e.g., abscess, tuberculous granuloma, parasitic infection, or radiation necrosis if the patient had received radiation treatment for a brain tumor). Conversely if a brain lesion appears solid on the MRI the main diagnosis would be either an HGG or a pseudotumoral demyelinating disease process such as multiple sclerosis. MRI examination suggesting a pseudotumoral nonneoplastic process would lead to further clinical tests to determine the type of process so that the appropriate therapy could be applied. However, if a neoplastic process is suggested from the MRI exam, then a stereotactic biopsy or surgical resection would be considered to determine whether the lesion is an HGG or metastatic lesion and used to determine whether further chemotherapeutic and/or radiation therapy treatments are needed. However, in many cases reliable differentiation of neoplastic from nonneoplastic brain lesions cannot be unequivocally made from conventional MRI. Proton MRS can help differentiate between brain tumors and nonneoplastic brain lesions. In two retrospective studies, discriminant function analysis using proton MRSI data correctly classified 78% of pediatric cases with primary brain lesions based on Cho/Cr ratio (the study included 32 subjects). In 69 adult patients with untreated primary brain lesions, proton MRS imaging correctly classified 84% of originally grouped cases based on NAA/Cho, NAA/Cr, and relative NAA and Cho levels (compared to the contralateral hemisphere). Combining proton MRSI and perfusion MRI, sensitivity of 72% and specificity of 92% were achieved using cutoff points corresponding to the diagnosis of tumor of 0.61 for the ratio of NAA/Cr and 1.5 for rCBV.
Recently the role of routinely used advanced MRI in differentiation of intracranial masses in adults was evaluated and the accuracy of an MRI-based strategy to differentiate among histologically confirmed neoplastic and nonneoplastic lesions was assessed. This review and assessment led to the development and evaluation of a MR-based decision paradigm that could be used to differentiate neoplastic from nonneoplastic processes. This “practical MRI-based” paradigm proposed in Figure 17-15 used results from postcontrast Gd-enhanced, DWI, PWI, and multivoxel H1-MRSI with well-defined thresholds to determine how well this multiparametric approach could not only accurately differentiate neoplastic from nonneoplastic lesions but also classify the type of neoplastic lesion (e.g., glioma from lymphoma and metastatic lesions) and nonneoplastic processes (e.g., abscess from tumefactive process). The MR-based diagnostic paradigm was evaluated in 40 patients who had complete data from all MR techniques to differentiate between tumors and nonneoplastic pseudotumoral processes. The accuracy, sensitivity, and specificity of the paradigm using the threshold values used in the ROC curves for each technique was found to be 90%, 97%, and 67%, respectively. For discrimination of high-grade from low-grade gliomas, the accuracy, sensitivity, and specificity was 90%, 88%, and 100%, respectively, and 85%, 84%, and 87% for discrimination of high-grade tumors and lymphomas from low-grade tumors and nonneoplastic pseudotumoral processes. The results of this paper clearly indicate that integration of advanced complementary MRI techniques with conventional anatomic MRIs can improve the diagnosis and classification of brain lesions, which in turn will add value to the clinical decisions being made for patients with these types of lesions (see Fig. 17-15 ).
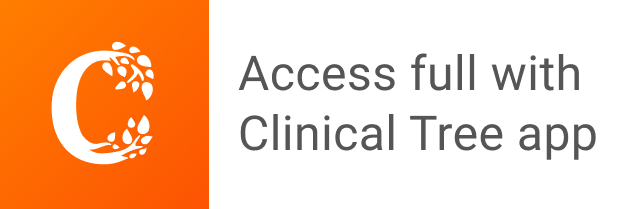