141CHAPTER 10
Brain Radiosurgery
As described in earlier chapters, stereotactic radiosurgery (SRS) represents a modern form of extremely precise radiation delivery, engaging alternative radiobiological mechanisms of tissue ablation. In contrast, conventional radiation therapy is administered in a highly fractionated schedule to deliver a desired cumulative target dose, at an acceptable therapeutic ratio. By refining the technology involved in target delineation, treatment planning, setup reproducibility, and quality assurance, radiation oncologists are now able to escalate the dose per fraction in a safe and effective manner. This has allowed the delivery of a biologically equivalent dose over a significantly abbreviated time course, while minimizing normal tissue toxicity.
The term “radiosurgery” was first conceived by Lars Leksell (Stockholm, Sweden) in 1951 (1). Prior to this time, conventionally fractionated intracranial radiation permitted a full dose of radiation to reach sensitive normal brain tissue, increasing the early and late toxicity profiles. Through the application of stereotactic neurosurgical techniques with rigid skull fixation, Leksell began targeting benign intracranial conditions and functional neurological disorders with sharp dose gradients of focused radiation. This achievement is especially remarkable, considering how much this technique predated the advent of CT and MRI. Once feasibility was proven, the approach evolved to the treatment of additional benign and malignant brain tumors and disorders.
Today, SRS continues to have a central role in the management of a variety of intracranial conditions. Multiple treatment platforms currently exist, including frame-based cobalt-60 platforms (Gamma Knife, Elekta Instruments, Atlanta, Georgia) as well as several linear accelerator–based units (CyberKnife—Accuray Inc., Sunnyvale, California; TrueBeam/Trilogy—Varian Medical Systems, Palo Alto, California; as well as others). All of these rely on an accumulation of multiple opposed beams to create a highly conformal target dose distribution. No current literature suggests a clear therapeutic advantage to any of the above-mentioned platforms (2). This chapter presents a concise summary of applications of SRS in the brain, including the evaluation, delivery, expected outcomes, and considerations involved in each condition. Readers are introduced to the management of benign (arteriovenous malformation, acoustic neuroma, meningioma, pituitary adenoma, vagal schwannoma) and malignant (both primary and secondary) brain lesions, as well as functional radiosurgery (trigeminal neuralgia, thalamotomy, pallidotomy). This chapter is meant to highlight the important steps of patient selection, treatment planning, safety considerations, and expected outcomes.
ARTERIOVENOUS MALFORMATION
Introduction
Intracranial arteriovenous malformation (AVM) represents an anomalous communication (nidus) between the arterial and the venous system 142devoid of a capillary network. With an incidence of approximately 1/700 in the United States (3), AVMs place patients at a 1% to 5% annual risk of hemorrhage (4). These hemorrhages can be fatal or neurologically devastating in a number of instances. The incidence of a fatal hemorrhagic event has been reported to be as high as 10% to 20% as a result of exposure of the venous system to an abnormally high pressure (5). In addition, AVMs can cause epilepsy and medically refractory headache disorders.
Contemporary treatment options include observation, surgical resection, embolization, SRS, or a combination thereof. Even when discovered in the asymptomatic setting, patients are often encouraged to pursue therapy because of the devastating implications of a hemorrhagic event. When feasible, surgical resection remains the primary treatment approach, and allows immediate removal of the abnormal vascular focus. Postoperative morbidity and mortality risk is reliant on factors including size, location, and pattern of venous drainage, and can be scored using the Spetzler–Martin grading system I to V (6). The ideal surgical candidates (score I) are those with a small superficial lesion in a noneloquent location. Should a patient present with a more surgically challenging lesion (especially grades 4 or 5), or if the patient is a poor candidate for general anesthesia, the therapeutic discussion widens to include consideration of embolization or SRS.
Patient Selection
Should a patient with AVM present with a small (<3 cm), deep, inoperable focus of disease, SRS represents an effective and safe treatment alternative to surgery/embolization. By delivering a high dose of conformal radiation, SRS prompts a prolonged reaction that often results in the occlusion of the vascular abnormality. Specifically, a cytokine-driven process results in endothelial cell proliferation, thrombosis, and obliteration of the AVM nidus termed “endarteritis obliterans.” This reaction can take approximately 1 to 3 years before obliteration is complete.
The obliteration response rates of AVM with SRS have been reported between 75% and 90%, and are dependent on multiple factors. Several institutions have reported a relationship between nidus volume and successful obliteration of an AVM. Steiner et al. reported on the Swedish experience after treating 945 AVMs. They reported an average volume of 2.1 cm3 for successfully obliterated lesions, and an average volume of 5.3 cm3 for nonobliterated cases. Mendenhall et al. reported on the Florida experience and the relationship of nidus volume to successful obliteration outcome. Posttreatment follow-up with MRI and arteriogram suggested an 81% obliteration rate in lesions measuring 1 to 4 mL, 89% between 4 and 10 mL, and 69% in lesions greater than 10 mL in volume. Colombo et al. reported on the Italian experience and reported an obliteration rate of 96% in small (<1.5 cm) lesions, and 33% in large (>2.5 cm) lesions. Several authors caution direct conclusions on the basis of volume–outcome data, citing that a larger AVM nidus may complicate interpretation of angiography, and make complete nidus coverage more challenging.
Figure 10.1 includes a proposed algorithm for navigating therapeutic options in the setting of AVM proposed by the International Radio Surgery Association (IRSA). This summarizes the above-mentioned treatment decisions (included in the following text).
Dose
Multiple retrospective series have reported on the appropriate therapeutic dose for AVMs, as well as the threshold for unacceptable treatment-related toxicity. Flickinger et al. reported the University of Pittsburgh experience of 197 patients with AVM treated with Gamma Knife SRS, and reported a significant relationship of the minimum target dose (Dmin) and the likelihood of nidus obliteration (7, 8). A sigmoid dose–response curve was generated demonstrating in-field obliteration rate on the basis of Dmin, including an obliteration rate between 70% and 98% on the basis of Dmin of 15 and 25 Gy.
Multiple groups have also reported on their experience of toxicity following SRS for AVM. The Pittsburgh group published an overall rate of 9% of neurological sequelae post-SRS (any neurological problem including headache). Of these patients, 58% had resolution of symptoms within 27 months. Dmin impacted resolution rate, with 89% resolution in patients with Dmin less than 20 Gy versus 36% resolution in patients with Dmin greater than 20 Gy. This group also reported that nidus location, as well as volume of normal brain tissue receiving 12 Gy, significantly affected the incidence of permanent post-SRS injury (9–11). Finally, a published dose–response curve demonstrated that the probability of obliteration reaches a maximum level at a marginal dose of approximately 23 Gy (12).
143
FIGURE 10.1 IRSA intracranial AVM management algorithm.
AVM, arteriovenous malformation; IRSA, International RadioSurgery Association.
With this in mind, the authors recommend a dose of between 16 and 24 Gy in a single-fraction linear accelerator (linac)-based setting or 21 and 23 Gy prescribed to the 50% isodose line in frame-based radiosurgery to optimize nidus obliteration, and minimize the risk of posttreatment toxicity.
Treatment Planning
The radiographic evaluation of an AVM typically includes a combination of imaging modalities including CT, computed tomography angiography (CTA), MRI, magnetic resonance angiography (MRA), or catheter angiography. CT scans assist in the detection of hemorrhage, and CTA assists in evaluating vascular detail, whereas MRI/MRA allows the best assessment of the surrounding brain parenchyma. Angiography is the most accurate method of categorizing the extent of aneurysms, obstructions, or outflow abnormalities that may exist prior to treatment, and allows grading of the AVM on the basis of Spetzler–Martin scale. Of note, to most accurately define intracranial structures, an image slice thickness of 0.1 to 0.15 cm is preferred.
Effective target delineation for SRS requires a clinician to exploit the capabilities of multimodality imaging modalities simultaneously. To accomplish this, a fusion of multiple image sets, typically from multiple imaging modalities, must take place within the treatment-planning software. Frequently, a team approach involving dosimetrists, medical physicists, and physicians is required to accurately align multiple image registrations to categorize target/normal tissue. This fusion can be accomplished in a manual fashion as users visually align patient rotation/translation, or automatically via computer-matching algorithms.
Once all the appropriate images have been fused within the treatment-planning software, clinicians begin to contour the appropriate treatment volume as well as critical normal tissue. A typical AVM plan will include a gross tumor volume (GTV) that encompasses the AVM nidus. Critical neighboring intracranial structures are also contoured as needed, including the brainstem, optic pathway, cochlea, and normal brain tissue. We recommend limiting the maximum dose to each structure over one fraction, including maximum doses of 15 Gy to brainstem, 8 to 10 Gy to optic pathway, and 4 Gy to cochlea, and minimizing the volume of normal brain tissue receiving 12 Gy dose.
Following the completion of contouring, and the prescription of appropriate dose targets and dose 144constraints, an SRS plan is created. This involves either a large number of stationary beams or fluid treatment arcs, to achieve a conformal high dose volume and rapid dose gradient. Beam-shaping techniques include the use of multileaf collimators (MLCs). An ideal plan accomplishes greater than 90% target coverage by prescription dose. Once approved, on-board imaging is used to verify patient setup prior to delivery of treatment. Figure 10.2 demonstrates each phase of treatment planning for an AVM case, as well as posttreatment follow-up imaging.
Outcomes
As mentioned earlier, the expected obliteration response rate for AVM following SRS is between 70% and 90% over 1 to 3 years. Colombo et al. reported an obliteration rate of 46% after 1 year and 80% after 2 years following treatment of 180 patients with AVM (13). Mendenhall et al. reported an overall angiographic cure rate of 80%, whereas Flickinger et al. reported a complete obliteration rate of 71%.
One disadvantage of radiosurgery for AVM as opposed to microsurgery is the risk of hemorrhage following treatment prior to AVM obliteration. However, multiple groups have reported a significant (but not complete) reduction in AVM bleeding risk following SRS. The Italian group reported a bleeding risk of 4.8% in the first 6 months following treatment, and 0% in 12 months following treatment. Maruyama et al. reported on a group of 458 patients from the University of Tokyo hospital, and demonstrated a bleeding rate of 5% during the latency period between SRS delivery and obliteration, and a bleeding rate of 6/250 (2%) after nidus obliteration (14). The Florida group noted a 4% rate of intracerebral hemorrhage after radiosurgery, with five-sixths of those patients suffering hemorrhage within 6 months posttreatment.
FIGURE 10.2 The angiography (A) and MRI (B) scan of a 61-year-old female with an AVM treated with definitive GK-SRS at a prescribed dose of 20 Gy at the 50% isodose line. Shown is a 10-shot GK-SRS plan (C) targeting the GTV. An MRI 2 years after treatment demonstrates significant treatment response (D).
AVM, arteriovenous malformation; GK-SRS, Gamma Knife stereotactic radiosurgery; GTV, gross tumor volume.
145Follow-Up
Once SRS is complete, the authors recommend close patient follow-up, typically on a 3-month and 6-month interval for the first year, with abbreviation in the event of clinical deterioration. Angiographic follow-up can be performed within 6 to 12 months following treatment, with the goal of complete nidus obliteration defined by Lindquist and Steiner. This includes normal circulation time, absence of former nidus vessels, and disappearance or normalization of draining veins (15). Clinical follow-up is encouraged even after nidus obliteration to ensure clinical stability and success.
ACOUSTIC NEUROMA
Introduction
Vestibular schwannomas (VSs; commonly referred to as “acoustic neuromas”) represent a benign intracranial neoplasm estimated to occur at an incidence of 0.8 to 1.0 per 100,000 patients per year in the United States (16). The majority of VSs occur in a sporadic and unilateral pattern. Patients with VS most frequently present with an incidentally discovered unilateral cerebellopontine angle mass, or gradually worsening unilateral hearing loss/tinnitus coupled with vertigo-like episodes or gait disturbance. Bilateral presentation is strongly associated with neurofibromatosis type 2 (NF2). The mass is typically located on the superior and inferior branches of the vestibular nerve, and is rarely found along the cochlear nerve. The average VS growth rate has been estimated between 1 and 3 mm/year, with approximately 5% of VS remaining stable or regressing during surveillance (17). Treatment options include observation, microsurgical excision, SRS or radiation therapy, with an expected local control rate that exceeds 90%.
Patient Presentation, Workup, and Selection
Patient-reported symptoms that arise from VS are direct sequelae of mass effect on neighboring cranial nerves. These include involvement of the acoustic nerve (sensorineural hearing loss/tinnitus), vestibular nerve (vertigo/gait instability), trigeminal nerve (facial paresthesias), facial nerve (facial paresis/taste change), and cerebellar peduncles (ataxia). In selected cases of large tumors, compression of the fourth ventricle may even lead to hydrocephalus.
Each patient with VS demands a thorough history and physical exam, baseline audiometric testing, as well as diagnostic imaging. The standard imaging modality for VS is a brain MRI with and without contrast. The lesion will typically enhance on T1-weighted images, and is frequently located in close proximity to the internal auditory canal. It is not uncommon for the canal to be dilated by the tumor, although some of these tumors are purely intracanalicular. Among other common cerebellopontine (CP) angle tumors, meningiomas generally do not have the same pattern involvement of the internal auditory canal, and epidermoid cysts look entirely different on all salient imaging sequences. In general, VS can be diagnosed based on MRI without histopathological correlation. A Kroos staging system was developed (Stages I–IV) based on size and extension to the nearby brainstem (18).
Given the benign nature of VS, as well as the high expected local control rate with treatment, the therapeutic discussion with patients should encompass two main goals: safe delivery of disease control and the limitation of cranial nerve deficits. No prospective randomized multi-institutional study has directly compared surgical excision with radiation therapy; however, retrospective single-institution series indicate similar oncological outcomes (19). Because of the higher risk profile with surgery, most centers prefer SRS or observation as the primary management strategy for small tumors. Large tumors, more symptomatic tumors, and tumors presenting with hydrocephalus generally require microsurgical resection. In addition, patients may be considered for surgical excision on the basis of age, location, serviceable hearing, and local expertise.
Several microsurgical approaches exist for excision of a VS, including the retrosigmoid approach, the middle fossa approach, or the translabyrinthine approach, each with differing rates of hearing preservation, facial nerve injury, and tumor accessibility on the basis of primary location (20–22). In addition to typical operative risks, surgery can place patients at risk of cerebrospinal fluid leakage and meningitis. A surgeon will consider baseline serviceable hearing function (minimal goal of >50% speech discrimination at 50 dB), local anatomy, patient performance status, tumor size, and likelihood of a complete resection prior to electing one of the above-mentioned approaches.
Outcomes
Radiosurgery has been demonstrated to be an excellent alternative to microsurgery for VS, and is probably best considered as the standard of care in 146the modern era. Given the steep learning curve for microsurgery, the operative risks, and the benign nature of the disease, SRS often has a better ratio of reward to risk for these patients (23, 24). Multiple institutions have reported on comparable outcomes delivering both fractionated stereotactic irradiation (fSRT) and SRS (25). On the basis of the nature of this text, this chapter focuses on the outcomes of SRS.
As stated earlier, SRS represents a convenient and safe alternative to fSRT. Most institutions use SRS to deliver a one- to three-fraction course of precise radiation therapy to a mean dose between 12 and 18 Gy. On the basis of reported single-institution outcomes, local control is expected to surpass 90%, and expected long-term cranial nerve toxicity rates are less than 5% (26, 27). One of the initial studies involving Gamma Knife radiosurgery (GK-SRS) to treat VS was published by Noren et al. from the Karolinska Institutet in Sweden (26). They reported a local control (smaller or stable lesion) rate of 94%. Several other institutions have found similar results including University of Pittsburgh reporting a 5-year local control (smaller or stable lesion) rate of 97% (29), University of Virginia reporting a local control of 94% (30), and several others reporting 10-year local control rates ranging from 90% to 98% (31, 32).
The ability of SRS to deliver a steep dose gradient facilitates avoidance of substantial radiation exposure to critical nearby radiosensitive structures including cranial nerves, the cochlea, and the brainstem. When properly executed, this has translated into an extraordinarily safe toxicity profile as reported in the previously mentioned studies while delivering a high-dose/fraction course of SRS to a congested CP angle space. Noren et al. reported a 4% long-term facial weakness rate, and 77% hearing preservation rate. Flickinger et al. reported preserved long-term facial nerve and trigeminal nerve function rates above 98%. Others have also reported comparable cranial nerve toxicity rates between 1% and 5% (33).
Multiple single-institution reports have been published comparing outcomes of microsurgery and SRS in patients with VS. The largest is a French study reported by Regis et al., which included a total of over 200 patients with VS. This study demonstrated significant improvements in serviceable hearing preservation (40% vs. 5%), and less CN VII (0% vs. 47%) and CN V (4% vs. 29%) toxicity with SRS (34). Follow-up studies by Myrseth et al. and Pollock et al. have confirmed these findings with significant improvements in hearing preservation rates (between 63%–68% and 0%–5%) and less CN toxicity (<5% vs. 17%–26%) for patients undergoing SRS versus microsurgery (33, 34). In addition, the rare devastating microsurgical risks of death, meningitis, stroke, and anesthesia complications are generally not seen with SRS, increasing its appeal for patients with minimal symptomology.
Treatment Planning
The radiographic evaluation of VS for treatment planning often includes a combination of imaging modalities including CT and contrast-enhanced MRI. MRI allows the best assessment of surrounding brain parenchyma and soft-tissue structures including cranial nerves. Of note, to most accurately define intracranial structures for contouring, the authors recommend an image slice thickness of less than 0.2 cm, as well as minimal space between images.
Effective target delineation for SRS requires the clinician to capitalize on the capabilities of multiple imaging modalities simultaneously. To accomplish this, a fusion of multiple image sets from multiple imaging modalities must take place within the treatment-planning software. A team approach involving dosimetrists, medical physicists, and physicians is required to accurately align multiple image registrations to categorize target/normal tissue. This fusion can be accomplished in a manual fashion as users visually align patient rotation/translation, or automatically via computer-matching algorithms.
Once all the appropriate images have been fused within the treatment-planning software, clinicians begin to contour the appropriate treatment volume as well as critical normal tissue. A typical VS plan will include a GTV that encompasses macroscopic tumor visualized on CT/MRI. This structure will typically then be prescribed a dose of 12 to 13 Gy in one fraction, or 18 Gy over three fractions, via isodose line calculation (typically 50% Isodose Line (ISD) for GK-SRS and 70%–90% ISD for linac-based). Critical intracranial structures are also contoured including brainstem, eyes, optic nerve pathway, cochlea, as well as normal brain tissue. We recommend limiting the maximum dose to each structure over one fraction, including maximum doses of prescription dose to brainstem, 8 Gy to the optic nerve pathway, and 4 Gy to cochlea (for patients with serviceable hearing), and minimizing the volume of uninvolved brain tissue receiving 12 Gy dose.
Following the completion of treatment volumes, and prescription of appropriate dose targets and 147dose constraints, an SRS plan is created. SRS has been effectively delivered using multiple treatment platforms including frame-based, multi–cobalt-60 source GK-SRS, as well as linac-based platforms including each platform discussed in the introduction of this chapter. Linac-based plans can be formulated to involve either a large number of stationary beams or fluid nonopposed treatment arcs, to achieve a conformal high dose volume and rapid dose gradient. Beam-shaping techniques include the use of MLCs. An ideal plan includes a prescription dose coverage of at least 95% of the GTV, as well as rapid dose gradient at circumferential distances 2/5/10 mm away from target tissue. Once approved, onboard imaging is used to verify patient setup prior to delivery of treatment. Figure 10.3 demonstrates diagnostic imaging and treatment planning of an acoustic neuroma.
Follow-Up
As stated earlier, the growth rate of VS is quite prolonged. This mandates extended posttreatment follow-up with patients, as well as repeat MRI imaging at time points 6-, 12-, 24-, and 48-month posttreatment intervals. In addition, a transient posttreatment swelling or increased prominence of a VS may be observed prior to delayed regression. The multidisciplinary team must thus use clinical judgment to infer treatment response or disease progression. In addition, cranial nerve and neurological function must be monitored diligently.
MENINGIOMA
Introduction
An estimated 20% to 35% of primary intracranial neoplasms originate from the three layers of meningeal tissue that envelop the brain (36), and are thus termed “meningiomas.” The rate of diagnosis increases with age, and peaks in the fifth and sixth decades of life. Several risk factors have been proposed including female gender, genetics, hormone replacement (37), and prior intracranial radiation exposure (38). Although 80% to 90% of meningiomas are categorized as benign World Health Organization (WHO) Grade I lesions, the associated edema and mass effect they cause may result in significant patient morbidity. Aggressive variants 148have been reported with a higher propensity for invasion, recurrence, and rapid growth pattern. The current WHO grading schema is based on mitosis per high-power field (mit/hpf), with WHO Grade I (<4 mit/hpf) making up 80% of meningiomas, WHO Grade II (4–20 mit/hpf) making up approximately 10% to 20% of meningiomas, and WHO Grade III (>20 mit/hpf) being very rare and accounting for the remaining patients.
FIGURE 10.3 The MRI (A) scan of a 55-year-old male with a right-sided acoustic neuroma treated with definitive CK-SRS. Shown is a 72-field CK-SRS plan (C and D) targeting the GTV. An MRI 3 years after treatment demonstrates stability following treatment (B).
CK-SRS, CyberKnife stereotactic radiosurgery; GTV, gross tumor volume.
Patient Selection
A large percentage of WHO Grade I meningiomas are discovered incidentally on imaging. If discovered during an asymptomatic presentation, and as a small volume, many advocate for conservative management with surveillance serial imaging at 6-month intervals. If the lesion remains stagnant, serial yearly imaging is a reasonable management strategy. Once repeat scans demonstrate a significant growth in volume, or symptoms begin to develop, the therapeutic index of resection becomes more appropriate. Multiple surgical series have demonstrated that a macroscopic complete resection with excision of dural attachments and any abnormal bone yields the highest rate of local control of approximately 90% (39). Once the extent of surgery becomes limited by nearby critical structures, including neurovascular structures and location, the propensity to recur increases to as high as 40% to 50%. Once at a higher risk of local recurrence, combining surgery with a course of postoperative radiation is considered.
Radiosurgical Management
A number of clinical scenarios exist that would warrant the use of radiation in the management of meningioma. As mentioned earlier, once the maximum extent of surgery is a subtotal resection (STR), radiation may provide an improvement in local control of gross residual disease. Radiation may also replace definitive surgical therapy if a patient is deemed a suboptimal surgical candidate, or a lesion is found in an inaccessible/eloquent region of the brain. Additional indications include treatment of a recurrence following surgery. Each of these scenarios, as well as the data that support the indication of radiation, is discussed in the following text.
A number of single-institution series demonstrate both the feasibility and the efficacy of conventional external beam radiation to treat meningioma in the event of STR. The majority of these studies used a dose of approximately 54 Gy delivered over a 30-fraction course. The University of California–San Francisco reported on 140 patients with meningioma following an STR and showed a significant dose–response relationship above 52 to 53 Gy. This included an improvement in 10-year progression-free survival (PFS) of 65% versus 93% in benign lesions, and a 5-year PFS of 17% versus 63% in malignant meningiomas (40). The University of Florida also compared outcomes of observation with those of postoperative radiation in 132 patients with meningioma with STR, and reported an improvement in 10-year local control of 18% versus 82% (41).
When electing to treat via conventionally fractionated external beam radiation, the majority of radiation oncologists follow the protocol established by Radiation Therapy Oncology Group (RTOG) 0539, a phase II trial evaluating the appropriate dose of external beam radiation for patients with meningioma. This protocol first divides patients with meningioma into Group I (WHO Grade I with gross total resection [GTR] or STR), Group II (WHO Grade II with GTR or recurrent WHO Grade I), or group III (WHO Grade II with STR, recurrent WHO Grade II, and all WHO Grade III lesions). Patients in Group I are observed, with an initial report at the 2016 annual meeting of the American Society for Radiation Oncology (ASTRO) demonstrating a 5-year PFS of 94% for patients with GTR and 73% in patients with STR. Patients in Group II received 54 Gy in 30 fractions. The same report demonstrated a 5-year PFS of 85% in patients with WHO Grade II STR, and 73% in recurrent WHO Grade I lesions. Patients in Group III undergo 60 Gy in 30 fractions, with outcomes of this group yet to be reported.
With the advent of refined surgical techniques and tumor imaging, the predominant contraindication of surgical resection is proximity to critical structures. The ability of radiosurgery to dramatically reduce the dose of radiation delivered to normal structures has allowed radiation oncologists to safely and effectively treat lesions with discernable borders in unresectable locations with SRS. Multiple single-institution series have shown the use of radiosurgery to produce local control rates above 90% with median marginal doses between 12 and 13 Gy (42). This includes definitive treatment via linac-based or GK radiosurgery platforms. Reports have also demonstrated efficacy in challenging locations such as the cavernous sinus (43) and optic nerve sheath (44).
149Radiation Safety and Planning
Along with the above-mentioned high rates of local control and PFS, several studies have reported on the safety of radiation therapy in the treatment of meningioma. The University of Pittsburgh reported toxicity rates of new or worsened neurological symptoms at 9% in 99 patients treated via GK-SRS, with 96% of patients considering it a “success” on follow-up surveys (45). When the same group reported on GK-SRS treatment of cavernous sinus meningiomas, they reported a 91% rate of improved or stable neurological status posttreatment. Hakim et al. reported on outcomes following linac-based SRS to 155 meningiomas. They reported a 4.7% rate of permanent complications because of radiosurgery at a median of 10.3 months.
Effectively delivering a course of SRS-appropriate targeting of the lesion is critical. The standard imaging of choice remains a contrast-enhanced brain MRI. CT imaging may assist in identifying hyperostosis of involved bone, as well as involvement of the skull base. Controversy exists regarding the margin, as well as the necessity of including a dural tail within treatment volumes. Many SRS trials to date have used margins on the order of 2 to 3 mm, and largely omitted the dural tail. Reports indicate that, especially in the setting of WHO Grade II meningioma, an overly conformal treatment volume may precipitate a higher risk of local recurrence (46). Finally, adjacent hyperostotic bone has been shown on surgical series to have a significant rate of involvement, and is considered worthwhile to include, as it is a frequent site of relapse (47). With this in mind, we would recommend a single-fraction SRS course to include a prescription marginal dose between 12 and 14 Gy, encompassing grossly evident disease, hyperostotic bone, with at most a 2-mm expansion.
The ideal radiosurgery plan includes at least 90% coverage of the target with the above-mentioned dose, as well as limited dose to nearby critical structures. This includes single-fraction maximum doses below prescription dose to brainstem, 8 Gy to the optic nerve pathway, and 4 Gy to cochlea, and minimizing the volume of uninvolved brain tissue receiving 12 Gy dose. An example of a radiosurgery plan for meningioma is found in Figure 10.4.
Follow-Up
We recommend close follow-up following radiosurgery treatment. This includes a 1-month posttreatment visit to ensure there is no acute toxicity, followed by clinical evaluation at 6- to 12-month intervals, and yearly brain MRIs thereafter.
FIGURE 10.4 The pretreatment MRI (A) scan of a 59-year-old male with a large left-sided meningioma treated with definitive CK-SRS. Shown is an 81-field CK-SRS plan (B and D) targeting the GTV. An MRI 2 years after treatment demonstrates stability following treatment (C).
CK-SRS, CyberKnife stereotactic radiosurgery; GTV, gross tumor volume.
150PITUITARY ADENOMA
Introduction
Approximately 10% to 15% of primary adult brain tumors arise from the pituitary gland (48). Because of a central location and interconnected endocrine functions, tumors of the pituitary gland may present with a variety of symptoms. Mass effect on the optic pathway can result in classic bitemporal hemianopsia (“tunnel vision”), as well as compression of cerebrospinal fluid drainage causing obstructive hydrocephalus. Disruption of endocrine pathways can result in multiple syndromes related to inappropriate hormone production including Cushing’s adrenocorticotropic hormone (ACTH), acromegaly (GH), galactorrhea (prolactin), and hyperthyroidism (TSH). Although typically benign, slow growing, and limited in metastatic potential, the local physical and physiological effects of pituitary adenomas may become quite problematic for patients, and present a therapeutic challenge for physicians.
A select population of patients with incidentally discovered, nonsecretory microadenomas (<1 cm in size) of the pituitary gland are routinely observed. In addition, certain patients with microadenomas who present with oversecretion of prolactin may be managed medically with dopamine agonists such as bromocriptine. As these tumors become more physiologically active, or locally compressive, the standard of care in most centers is transsphenoidal microsurgery. Several reports have demonstrated that local control with surgery alone is excellent at approximately 90% following a complete surgical excision (49, 50), and hormonal control may range from 50% to 80% (51). The risk of local recurrence is approximately 40% to 50%. In the event of incomplete resection, consideration must be given to radiation delivered via standard fractionation or radiosurgery to optimize local control.
Workup and Management
The average pituitary gland measures approximately 0.8 to 1.5 cm in size, and is confined in the sella turcica. Within immediate proximity to the pituitary gland are the optic chiasm (anterosuperior) and the cavernous sinus (lateral). The appropriate modality of diagnostic imaging to categorize malignancies of this congested region is a multiplane MRI of the brain with gadolinium contrast obtained with a slice thickness and image interval of less than 0.2 mm.
Once a patient is considered for radiation, it is imperative that all imaging available, both preoperative and postoperative, be accurately fused within the radiation-planning system. This allows for the most accurate delineation of any residual malignant tissue and postsurgical changes. Previous treatment protocols using fractionated external beam radiation were able to achieve excellent local control; however, fields were inclusive of the entire hypothalamic–pituitary axis, and resulted in undesirable posttreatment hypopituitarism (52). SRS now allows the delivery of a higher dose per fraction with rapid dose falloff to optimize target dose, and minimize the dose of radiation to nearby critical structures. By integrating the benefits of stereotactic guidance, patient immobilization, precise delivery, and improved imaging, SRS has become a conventional approach to postoperative radiation of pituitary adenomas. Typical target volumes encompass a GTV of enhancing postcontrast tissue, with rapid dose falloff within 2 to 5 mm around the target. As these lesions usually are located within close approximation to the optic chiasm, extra attention is given to avoid single-fraction doses of greater than 8 Gy to the optic system. With this in mind, a fractionation schedule of two to five fractions may be necessary to accomplish a treatment plan with a safe optic chiasm dose. Figure 10.5 demonstrates a pituitary adenoma case treated with the above-mentioned parameters.
FIGURE 10.5 The MRI (A) scan of a 48-year-old male with a large pituitary adenoma treated with definitive GK-SRS. Shown is an 18-shot GK-SRS plan (B and C) targeting the GTV.
GK-SRS, Gamma Knife stereotactic radiosurgery; GTV, gross tumor volume.
151Outcomes
Several institutions have reported clinical outcomes using postoperative fractionation schedules ranging from 14 to 18 Gy for nonfunctioning adenomas, and 18 to 30 Gy for functioning adenomas. Local control rates from these reports surpass 90% (53, 54). Sheehan et al. reported that a marginal dose less than 12 Gy using GK-SRS resulted in suboptimal local control rate compared with doses between 12 and 20 Gy (55).
Surgical decompression remains the most rapid method of symptom improvement. As mentioned, the long-term rate of local control in the event of subtotal resection or medical inoperability remains excellent following the addition of radiation therapy. Van den Bergh et al. reported on outcomes of patients with subtotal resection of nonfunctional pituitary adenomas who underwent postoperative observation or radiation. This study reported a disease progression rate of 4% following radiation versus 57% disease progression following postoperative observation after subtotal resection (56). Of those patients with a recurrence after observation, 95% were able to attain local control with salvage radiation.
Stabilization of hormonal dysfunction following irradiation remains a challenge, with a wide range of outcomes reported to date. SRS has yielded hormone remission in approximately 50% of prolactinomas (57, 58), 60% to 70% of acromegaly (59), and 70% to 80% of Cushing’s disease (60, 61) on the basis of recent reports. This remission takes place on a delayed timeline, with a median time to improvement of 12 to 24 months. Frequently a multidisciplinary approach is required to achieve hormone goals, including surgical, radiation, and medical management.
Follow-Up
Following SRS treatment of pituitary adenomas, we recommend a short-interval follow-up at a minimum of 3-month intervals within the first year. This includes monitoring of hormone levels, and any indication of adverse reactions to radiation. As the growth rate of pituitary adenomas is quite slow, a follow-up brain MRI is typically scheduled 6 months after treatment, followed by 1-year intervals thereafter to monitor physical progress. In the event of posttreatment inflammation/pain/headaches or visual changes, the first step of management is typically steroids followed by radiographic evaluation.
PARAGANGLIOMA, VAGAL SCHWANNOMA, AND OTHER TUMORS OF THE PARAPHARYNGEAL SPACE
Introduction
Tumors of the parapharyngeal space are relatively rare and are probably best divided into schwannomas and other lesions. Schwannomas may arise from any nerve in the parapharyngeal space, including the sympathetic chain, and exhibit similar imaging characteristics to schwannomas in more common locations. These features include homogeneous contrast enhancement, easy delineation from surrounding structures, and intermittent cystic degeneration. Other tumors found in this location include paragangliomas, neurofibromas, and neurofibrosarcomas (62, 63). Paragangliomas, or glomus tumors, are uncommon, typically benign tumors that arise from the paraganglia of the autonomic nervous system. Although neuroendocrine in origin, these tumors are rarely secretory (64). Unlike schwannomas, these tumors typically exhibit a “salt and pepper” pattern of enhancement on contrast-enhanced images because of tumoral flow voids. Frequently discovered as a slow-growing, solitary, asymptomatic mass, these lesions usually present in the fourth and fifth decades of life. Their growth rate is slow (doubling time of approximately 4.2 years), with a malignancy potential of less than 5%. If amenable, the primary treatment modality for tumors of the parapharyngeal space is surgical excision, but radiosurgery is an excellent treatment alternative in selected cases.
Patient Selection/Evaluation
Once diagnosed with a parapharyngeal tumor, several factors affect a patient’s candidacy for definitive management via surgical excision or radiation therapy. This includes the extent of local invasion, size, location, proximity to sensitive/eloquent structures, overall medical condition, patient preference, and local expertise.
Typical evaluation includes a thorough cranial nerve examination, as well as a stereotactic brain MRI with gadolinium contrast. Scan slice thickness/interval must be less than 2 mm to accurately categorize the lesion and nearby anatomy. CT may also aid in the delineation of bony involvement. Vascular studies may assist in identifying angioarchitecture as well as vascular compression/compromise.
On the basis of published meta-analysis, the local recurrence/progression rate following either 152of these two treatment modalities is excellent and quite similar at approximately 2% to 3% (65). Although surgical intervention portends an excellent control rate, the risk of cranial nerve damage and deficits has approached 30% to 40% in surgical series (66, 67), so it can be argued that surgical intervention is ideal for larger tumors rather than for smaller or incidental masses.
Radiation Treatment
If deemed a suboptimal surgical candidate because of medical condition or tumor characteristics, a definitive course of radiation therapy has been demonstrated to provide an excellent and comparable rate of local control. As mentioned earlier, to create an accurate and effective radiation plan, a fusion of imaging modalities within the radiation delivery software is necessary. This allows the team to take advantage of superior abilities of each imaging modality while creating a treatment plan.
As these tumors are extremely rare, the majority of treatment recommendations and outcomes data are derived from single-institution experiences with limited volume case series. Multiple institutions have recently reported on the outcome of SRS used to effectively and safely treat vagal schwannomas. Chun et al. reported the University of Texas (UT) Southwestern experience treating 31 patients to a total dose of 25 Gy over five fractions, and documented a 100% local control rate, whereas 16% of patients had grade 1 toxicity, and one patient had grade 2 headaches (68). Pollock described the Mayo Clinic experience treating 42 patients with GK-SRS, using a mean marginal dose of 14.9 Gy, reporting a 98% rate of stable/decreased disease, and a 2% progression rate (69). They noted hearing preservation in 81% of patients at 4 years. The University of Pittsburgh reported a local control rate of 100% following SRS treatment of glomus jugulare tumors (70). Multiple European series have also demonstrated 95% to 100% radiographic stability/improvement following SRS (71, 72).
With these data in mind, we recommend an SRS dose of approximately 12 to 16 Gy over one fraction delivered with high conformality. This includes adequate target coverage (95% coverage with prescription dose) as well as rapid dose falloff within 2 mm (90% isodose) and 5 mm (50% isodose) outside the target volume. As described earlier, critical intracranial structures are also contoured including brainstem, eyes, optic nerve pathway, cochlea, as well as normal brain tissue. Our authors recommend limiting the maximum dose to each structure over one fraction, including maximum doses of prescription dose to brainstem, 8 Gy to the optic nerve pathway, and 4 Gy to cochlea, and minimizing the volume of uninvolved brain tissue receiving 12 Gy dose. Once approved, on-board imaging is used to verify patient setup prior to delivery of treatment.
BRAIN METASTASIS
Introduction
Overall, approximately 25% of cancer patients eventually develop brain metastasis, resulting in a significant negative impact on their long-term prognosis (73). Metastatic tumors to the brain outnumber primary brain malignancies at a rate of approximately 10 to 1 (74). The risk of developing secondary disease to the brain is directly related to the patient’s cancer histology, with tumors of the lung, breast, and melanoma demonstrating the highest propensity for central nervous system (CNS) spread (75, 76). As the sensitivity of diagnostic imaging improves, and systemic agents improve control of microscopic disease proximal to the blood–brain barrier, the incidence of brain metastasis continues to rise. On the basis of presenting symptoms, burden of intracranial disease, extracranial tumor control, performance status, and treatment history, treatment options can include surgical resection, whole-brain radiation therapy (WBRT), SRS, or supportive care. This chapter discusses the critical points of evaluation, therapeutic options, and outcomes in the management of a patient with intracranial metastatic disease.
Evaluation/Prognosis
Once a patient with a known cancer diagnosis presents with new neurological symptoms such as headache, strength/sensory changes, ataxia, altered mental status, seizure, nausea, or cranial nerve deficits, the clinical suspicion of brain metastasis must remain high until proven otherwise. In addition, many otherwise asymptomatic patients benefit from screening MRI scans of the brain during cancer staging. In either case, the diagnostic modality of choice is a contrast-enhanced brain MRI, preferably using a stereotactic protocol and slice thickness/image interval width less than 0.2 cm. Once metastatic disease is confirmed, optimal management includes a rapid relief of symptoms. This may include a temporary course of corticosteroids, typically an intravenous (IV)-loading dose of approximately 8 to 10 mg, followed by an oral 153maintenance dose of 4 to 6 mg every 6 hours alongside a prophylactic proton pump inhibitor. Antiepileptic medications are worthwhile only if the patient presents with seizure symptoms; otherwise, prophylactic antiseizure medication has demonstrated no benefit (77).
Multiple tools have been developed to accurately prognosticate patients with brain metastasis. Sperduto et al. created a disease-specific graded prognostic assessment (GPA) on the basis of the primary cancer and factors including age, performance status, and intracranial disease, and extracranial control. Following a multi-institutional analysis of over 4,200 patients with brain metastasis, a comprehensive point system was able to predict median overall survival between 3 and 18 months (78). Prior to this, Gasper et al. created a recursive partitioning analysis (RPA) on the basis of 1,200 patients with brain metastasis treated on three different RTOG trials between 1979 and 1993. On the basis of factors including performance status, age, and extracranial status, patients were grouped into classes I to III with median survival of 7.1 months in class I, 4.2 months in class II, and 2.3 months in class III (79, 80). Each of these tools assists physicians in discussing potential outcomes with patients, and has provided physicians with a helpful metric when debating treatment options.
TREATMENT MODALITIES/OUTCOMES
As discussed earlier, the optimal management of brain metastasis depends on several factors including the primary disease, patient presentation, performance status, intracranial and extracranial disease volume, and age. A multidisciplinary team including neurosurgeons, medical oncologists, radiation oncologists, neuroradiologists, and supportive care specialists may be needed to establish an appropriate treatment strategy. There should be a mutual understanding of the goals and expected benefits from treatment between the patient and his or her physician, with continuous reevaluation. In the appropriate setting, surgical resection/debulking, WBRT, SRS, and supportive care are acceptable and worthwhile treatment options.
Surgery
As brain metastasis represents an incurable progression of disease, with typically a limited long-term survival, the role of surgical resection of secondary brain lesions has been an issue of investigation. If a metastatic tumor has resulted in a significant neurological deficit, surgery provides an effective method of immediate debulking of the mass, preventing further decline and potentially facilitating neurological recovery. Most agree that patients most suitable for surgery include those with a single accessible metastatic lesion, otherwise well-controlled systemic disease, and relatively intact functional status. This cohort has grown with modern cytotoxic and targeted agents providing enhanced systemic control of disease.
Multiple well-cited randomized controlled trials have demonstrated a benefit of the addition of surgical resection to WBRT alone in patients with a single brain metastasis and Karnofsky Performance Status (KPS) greater than or equal to 70. A seminal paper by Patchell et al. demonstrated multiple benefits with the addition of surgery to WBRT (36 Gy/12 fractions). This included a significant improvement in overall survival (40 weeks in the combined modality group vs. 15 weeks in the WBRT-alone group), local failure (20% vs. 52%), time to neurological death (62 weeks vs. 26 weeks), and prolongation of KPS greater than or equal to 70 (81). A Dutch study by Noordijk et al. confirmed a median survival benefit by adding surgery to WBRT (10 months vs. 6 months), particularly in those with stable extracranial disease (12 months vs. 7 months; 82).
Whole-Brain Radiation Therapy
Prior to the improvement of surgical technique and expanded feasibility, WBRT was used as a standard treatment approach to control macroscopic and suspected microscopic disease throughout the brain. With the advent of effective and safe surgical excision, the role of WBRT was called into question. Patchell et al. performed a prospective study evaluating surgery alone versus surgery plus WBRT in patients with single brain metastasis and KPS greater than or equal to 70. They reported an improvement in both local control (90% vs. 54%) and intracranial control (72% vs. 30%), as well as less neurological death (14% vs. 44%), with the addition of WBRT to surgery alone (81). There was no difference in overall survival. European Organization for Research and Treatment of Cancer (EORTC) 22952 affirmed these findings when comparing local therapy (either surgery or SRS) alone or in combination with WBRT in 353 patients with one to three metastases, stable extracranial disease, and intact performance status. The authors demonstrated a significant improvement in 2-year local control (73% vs. 41%) and 2-year intracranial 154control (77% vs. 58%), with no change in overall survival (83).
Most would agree today that the use of WBRT is reserved for postoperative patients with progressive disease, or inoperable patients with diffuse and numerable disease burden. In general, we recommend WBRT for patients with greater than 10 intracranial lesions, a large volume of involved disease, or leptomeningeal carcinomatosis.
Stereotactic Radiosurgery
WBRT provides physicians the ability to target intracranial metastasis with a noninvasive approach, while controlling extracranial disease with systemic therapy. However, conventionally fractionated WBRT permits a full dose of radiation to reach a large volume of sensitive uninvolved brain tissue, increasing the early/late toxicity profile. By combining techniques similar to those in stereotactic neurosurgery, and taking advantage of the relative immobility of the brain, SRS was implemented to provide a high dose per fraction of radiation to a discernable focus of disease, and spare nearby normal tissue with rapid dose gradients.
Several studies have demonstrated the efficacy of SRS as a definitive therapy, and in combination with WBRT or surgical resection. When delivered following surgery, multiple single-institution series have reported local control rates between 70% and 90%, whereas the rate of intracranial failure remained approximately 25% to 50% (84–86). RTOG 95-08 compared WBRT (37.5 Gy/15 fractions) with WBRT plus SRS for patients with one to three brain metastases. In patients with a single unresectable focus of disease, the addition of SRS provided an improvement in median survival from 4.9 to 6.5 months (p = .039), as well as improved performance status and less steroid dependence (87–89). When used alone, SRS provided excellent local control rates between 70% and 90%; however, intracranial failure remained at approximately 50%. The Mayo Clinic published a single-institutional series directly comparing SRS (n = 23 patients) with surgical excision (n = 74 patients), with no difference in overall survival, but a local recurrence rate of 58% with surgery versus 0% with SRS (90).
On the basis of the existing literature, SRS remains an effective and accepted treatment approach to patients with disease burden/location that is not amenable to complete surgical excision, or patients who either wish to avoid surgery or are medically inoperable. Reports indicate that SRS may be safely used for patients presenting with up to 10 brain metastases (91), and that overall disease volume may be more relevant than absolute lesion number. Our authors will generally recommend SRS for lesions up to 4 cm in size in nonsurgical candidates, on the basis of location and the overall case presentation.
SRS Dose Schedule/Treatment Planning
Most SRS centers follow the dose/fractionation schedule generated by RTOG 9005. This phase I study demonstrated safe, single-fraction radiosurgery doses of 24 Gy to lesions less than or equal to 2 cm, 18 Gy for lesions of 2 to 3 cm, and 15 Gy to lesions of 3 to 4 cm (92). A typical linac-based SRS plan will include 100% coverage of GTV to an 80% isodose line, with the 90% isodose line covering at least 50% of the GTV. Prescription dose will then be to the 80% isodose line, generating a central dose of 1.1 to 2 times the prescription dose. Once coverage is adequate, the goal is to promote conformity of dose. Our center uses rings 2, 5, and 10 mm from the target to set goals of less than 50% isodose line by a 10-mm distance. Figure 10.6 demonstrates a representative SRS plan targeting metastatic breast carcinoma in the brain.
FIGURE 10.6 The MRI (A) scan of a 53-year-old female with intracranial metastatic breast carcinoma treated with definitive CyberKnife stereotactic radiosurgery. A total of six lesions were treated in this plan; shown is a 178-field CK-SRS plan (C and D) targeting the GTV. An MRI 1 year after treatment demonstrates significant interval treatment response (B).
CK-SRS, CyberKnife stereotactic radiosurgery; GTV, gross tumor volume.
155Follow-Up
As mentioned earlier, patients with SRS remain at a significant risk of intracranial failure when enrolled in a protocol that avoids WBRT. In addition, the protective effect of WBRT with regard to remote intracranial failure is relatively short-lived. With this in mind, our authors recommend a short-interval close follow-up with repeat contrast-enhanced brain MRIs on a schedule of every 3 months for the first year, and gradually expanded timecourse thereafter. In the event of recurrence, or new disease, the evaluation process must renew, with consideration to the benefit of each treatment modality and the current state of extracranial disease.
HIGH-GRADE GLIOMA (ANAPLASTIC GLIOMA/GLIOBLASTOMA MULTIFORME)
Introduction
High-grade gliomas include WHO Grade III (anaplastic astrocytoma, anaplastic oligodendroglioma, and anaplastic oligoastrocytoma) and WHO Grade IV (glioblastoma multiforme [GBM]) lesions. High-grade gliomas account for approximately 40% to 50% of primary adult brain lesions (93). The majority (75%–80%) of high-grade gliomas are of WHO Grade IV GBM histology. The criteria to distinguish these entities include histopathological detection of nuclear atypia, mitoses, endothelial cell proliferation, and necrosis. Several recursive partitioning analyses have been performed that allow physicians to prognosticate patients on the basis of age, performance status, extent of resection, and mental status (94, 95). On initial presentation, it is critical to distinguish histology, as the median survival for GBM ranges from approximately 9 to 20 months, whereas the median survival for astrocytoma can reach as high as 60 months. In addition, cellular markers including O(6)-methylguanine-DNA methyltransferase (MGMT) promoter methylation, telomerase reverse transcriptase (TERT), 1p19q codeletion, and isocitrate dehydrogenase (IDH) 1 or 2 mutation have all been reported as both prognostic and predictive for patients with malignant glioma, and are an increasingly important element of patient evaluation and management.
Patient Selection/Management
To date, the first step of therapy for a high-grade glioma is a maximum safe resection. The extent of resection (gross vs. subtotal) has been associated with improved median survival outcomes in several studies (96). Several autopsy series have demonstrated that approximately 80% of tumor recurrences are within 2 cm of the initial unifocal tumor bed. With this in mind, multiple studies have demonstrated that combining maximal safe resection with standard external beam radiation therapy (EBRT) provides a significant survival benefit to patients with malignant glioma (97, 98). If a tumor cannot be safely resected, then stereotactic biopsy is necessary to establish a diagnosis prior to definitive treatment.
The data regarding the benefit of radiation in combination with chemotherapy can be parsed out on the basis of histology. For patients with WHO Grade III Disease (Astrocytoma), the two major trials demonstrating a benefit to the combination of radiation and chemotherapy include RTOG 94-02 (99) and EORTC 26951 (100). Both studies compared postoperative radiation alone with postoperative radiation plus a procarbazine/CCNU/vincristine (PCV) regimen. Both studies established 1p19q/IDH1 as strong prognostic factors, and that the addition of chemotherapy significantly improved progression-free survival. Both of these studies also demonstrated an overall survival benefit of chemotherapy alone in patients with 1p19q codeletion, but failed to demonstrate a survival benefit in patients without this mutation. Further trials have demonstrated no significant oncological outcome difference between PCV and temozolomide (TMZ) regimens; however, TMZ has an improved hematologic/neuropathy/transaminitis toxicity profile. These data have been supplemented by recent published results of the CATNON trial (NCT00626990), which randomized patients with anaplastic glioma without the 1p19q codeletion to postoperative radiation alone versus postoperative radiation plus concurrent/adjuvant TMZ. This trial reported a significant 5-year overall survival (44% vs. 55%) and median progression-free survival (19 months vs. 42 months) improvement in patients who received adjuvant TMZ, advocating for adjuvant chemotherapy in those with 1p19q codeletion.
For patients who present with WHO Grade IV Disease (GBM), the seminal trial demonstrating a survival benefit to combination of radiation and chemotherapy was EORTC-NCIC trial 26981-22981 published by Stupp et al. (101). This trial randomized patients greater than 70 years of age with strong performance status to postoperative radiation therapy (60 Gy in 30 fractions) with or 156without concurrent and adjuvant TMZ. The authors demonstrated a significant 5-year overall survival benefit that was greatest in patients with MGMT promoter methylation.
Each of the trials mentioned in this chapter was performed with conventionally fractionated EBRT. With the advancement of radiation delivery techniques, many have questioned the potential role of definitive or additive radiation via SRS as a boost for selected patient groups. To date, there have been no prospective trials that have demonstrated a significant benefit of SRS boost in combination with standard surgical resection and conventional fractionated EBRT for primary malignant glioma. RTOG 93-05 randomized 203 patients with GBM to postoperative SRS followed by standard protocol EBRT plus systemic therapy versus standard protocol chemoradiation therapy alone. This trial reported no significant difference in median survival (approximately 13 months) between the two groups (102). Since that time, the ASTRO has published a review statement that SRS boost “does not confer a benefit in terms of overall survival, local brain control, or quality of life as compared with external beam radiotherapy and BCNU alone” (103). This review also went on to cite an insufficiency of evidence regarding the benefits/harms of using SRS at the time of initial presentation, progression, or recurrence for patients with malignant glioma. Therefore, as a general rule, the use of adjuvant SRS for high-grade glioma should be reserved for selected cases in which the patient has already received an adequate course of conventional EBRT.
Multiple single-institution and multicenter retrospective reviews have reported the use of SRS for patients with new/recurrent high-grade glioma (104–106). Each has shown potential improvements in outcome compared with historical controls; however, these reports must be interpreted cautiously because of a significant risk of selection bias. Multiple single-institution reports have demonstrated the potential safety and efficacy of concurrent SRS and bevacizumab in recurrent malignant gliomas. Together they have reported low incidence of grade 3 toxicity (107, 108), and improved outcomes compared with historical controls (109).
The University of Pittsburgh Cancer Institute reported on the treatment of 11 patients with recurrent GBM with GK-SRS to a median margin dose of 16 Gy alongside bevacizumab, and compared outcomes with 44 case-matched controls who underwent GK-SRS alone (110). This report demonstrated that the combination of GK-SRS and bevacizumab yielded a significant improvement in 1-year progression-free survival (15 months vs. 7 months) and overall survival (18 months vs. 12 months) compared with GK-SRS alone. Figure 10.7 demonstrates the MRI findings of patients from this trial.
Others have cautioned against the use of highly conformal radiosurgery in high-grade glioma, as the disease has an infiltrative growth pattern with borders that are difficult to distinguish, increasing the risk of a potential treatment miss. The previously cited experiences have treated lesions less than 3 cm in diameter with a single fraction (13–18 Gy), whereas lesions sized 3 to 5 cm in diameter received doses of 25 to 30 Gy in five fractions. With this in mind, our authors do not recommend the use of SRS in the up-front treatment of high-grade glioma, and reserve SRS for patients in the recurrent disease setting with low-volume disease.
FIGURE 10.7 The MRI (A) scan of a patient with a recurrent glioblastoma multiforme 4 months after upfront resection, conventional radiation, and temozolomide. (B) An MRI 1 year after salvage GK-SRS and bevacizumab. (C) The MRI of a patient with a recurrent left temporal lobe glioblastoma multiforme after resection, conventional radiation, temozolomide, and Novocure therapy. (D) An MRI 18 months after salvage GK-SRS and bevacizumab.
GK-SRS, Gamma Knife stereotactic radiosurgery.
157FUNCTIONAL RADIOSURGERY—TRIGEMINAL NEURALGIA, THALAMOTOMY, PALLIDOTOMY
Introduction
Functional SRS shifts the target of radiation from a discernable tumor or other anatomic lesion to a nonmalignant abnormal physiological pathway that has resulted in significant detrimental symptoms for a patient. These symptoms may include facial pain, intractable seizures, and essential parkinsonian tremors. Typically in each presentation, the patient has been refractory to medical management, presents as a suboptimal operative candidate, or has failed multiple additional treatment modalities.
Trigeminal Neuralgia (TGN)
The most widely used application of functional SRS is control of medically refractory trigeminal neuralgia pain. Trigeminal neuralgia is a symptomatic disorder of cranial nerve V that results in sudden and severe stabbing/shocking pain felt in a unilateral distribution of the affected nerve root (111). The International Headache Society defines classical trigeminal neuralgia (aka “idiopathic/tic douloureux”) as including paroxysmal pain attacks that are intense, precipitated by trigger areas, with no additional neurological deficits or disorders. The etiology is suspected to be an aberrant vascular compression of the affected nerve before it enters the brainstem. Initial standard of care includes a regimen of antiepileptic medication, with systemic review demonstrating symptom control in 90% of patients (112). Medication alternatives include open surgery with microvascular decompression, rhizotomy with glycerol injection or balloon decompression, or radiofrequency injury to the nerve (113). Several groups have published high rates of pain control with microvascular decompression surgery, which remains the gold standard of care for medically refractory cases. Barker et al. published a surgical series of 1,185 patients and documented 82% of patients achieving immediate pain relief, which was sustained as complete pain relief in 75% of patients at 1 year, and 64% of patients at 10 years (114).
SRS for Trigeminal Neuralgia
Retrogasserian radiosurgical rhizotomy is a noninvasive and safe procedure suitable for patients regardless of treatment history (115, 116). Multiple series have demonstrated the efficacy of SRS in the treatment of idiopathic, medically refractory, trigeminal neuralgia pain. Kondziolka et al. reported outcomes on 220 patients treated with a median dose of 80 Gy, and documented complete pain relief in 65% of patients at 6 months, 70% of patients at 1 year, and 75% of patients at 33 months (117). This group also cited improved pain relief in patients with younger age, fewer prior procedures, and increased risk of complications (numbness or paresthesias) with increased length of nerve irradiated (118). Multiple additional single-institution reports have published long-term “excellent pain control” rates of approximately 60% to 90% (119, 120). Although overall response rates are not as durable as for MVD, SRS for TGN allows the patient to avoid the risks unique to craniotomy, including anesthesia complications, hemorrhage, cerebrospinal fluid (CSF) leak, and infection.
SRS Technique
The majority of published data using SRS for TGN have been via the GK-SRS platform, according to previously described setup protocols. Treatment is typically prescribed to either one or two isocenters (separated by 3–5 mm), with a maximum dose of approximately 70 to 80 Gy, and a 50% isodose line as the nominal treatment volume. This is typically delivered using 4-mm-diameter collimators. One isocenter is always placed close to the root entry zone of the retrogasserian trigeminal nerve, with the 50% isodose volume outside the brainstem, and the 20% isodose volume abutting the brainstem. Single-institution studies have shown that dose rate, treatment time, and collimator size are not significantly associated with control rate or degree of pain relief (121, 122). Similar control rates and toxicity profiles have been reported with linear accelerator–based SRS platforms (123, 124). An example of GK-SRS–based radiosurgery is shown in Figure 10.8.
Thalamotomy/Pallidotomy
Parkinsonian tremors, rigidity, and akinesia remain a therapeutic challenge for neurologists and neurosurgeons. These symptoms are felt to originate from irregularities between activation and negative feedback mechanisms between the basal ganglia, caudate nucleus, and cerebellum that control voluntary and involuntary movements. Initial management strategies have included the use of dopamine agonists that actively cross the blood–brain barrier, including levodopa. Reports have demonstrated the efficacy of surgical pallidotomy/thalamotomy and deep brain stimulation (DBS) to alleviate the aforementioned symptoms in patients who become medically refractory (125–127). Durable efficacy from these procedures has reached 90% in some reports while using electrophysiological target localization, but is associated with the risks of an intracranial invasive procedure.
158
FIGURE 10.8 The MRI planning scan of a 70-year-old male with trigeminal neuralgia. A single-shot plan was delivered via GK-SRS, using 4-mm collimators. The dose prescribed was 42.5 Gy at the 50% isodose line.
GK-SRS, Gamma Knife stereotactic radiosurgery.
SRS and Parkinson’s Disease
For medically-refractory patients deemed suboptimal surgical candidates for DBS, either because of comorbidities or advanced age, noninvasive tissue ablation via SRS may be the best option for treatment. Although the therapeutic effects of SRS are delayed in comparison to surgery (approximately 4–8 weeks), some have reported symptom control efficacy rates of 90%, which is comparable to historical surgical controls (128). The Jefferson group performed pallidotomy or thalamotomy via SRS on 17 patients with medically refractory movement disorders and noted a clinical response and a ring-enhancing radiosurgical “scar” in the majority of patients approximately 4 weeks after treatment (129). Additional reports have documented significant improvements in patient-reported tremors and motor function scores after SRS-mediated ablation (129, 130).
Delivery of thalamotomy/pallidotomy via GK-SRS requires a multidisciplinary approach between neurologist, neurosurgeons, and radiation oncologists.
When performing a thalamotomy for tremor, the target is the ventral intermediate thalamic nucleus. The Swedish group recommends a target that measures 3 to 4 mm in the anteroposterior dimension, and is located 2 to 3 mm anterior to the midcommisural point, 22 mm lateral to midline, and 6 mm below the commissural plane. When performing a pallidotomy, the target includes the globus pallidus interna (GPI), which is immediately lateral to the internal capsule, superior to the optic tract, and posterior to the mammillary bodies, 2 to 3 mm anterior to the midcommissural point, and 4 to 6 mm inferior to the intercommissural line (131). An example of this is shown in Figure 10.9.
Treatment planning via GK usually involves placing a single isocenter, and using a 4-mm-diameter collimator, while prescribing a maximum dose between 120 and 140 Gy. The target is localized via pretreatment MRI studies obtained after placement of a stereotactic frame. Regardless of the therapeutic goal of radiosurgery, our authors recommend close-interval follow-up between radiation oncologists, neurologists, and neurosurgical services to monitor for response, reaction, and recovery following treatment. As discussed, the full symptomatic benefit of radiation may take weeks to develop.
FIGURE 10.9 The MRI planning scan (A) of an 85-year-old female with an essential tremor that underwent functional stereotactic Gamma Knife radiosurgery. Her treatment involved delivery of a single-shot plan, prescribed to 65 Gy at the 50% isodose line; two treatment sectors were blocked. An anterior commissure/posterior commissure (AC/PC) line was created for a frame of reference (B), and a 1-year posttreatment MRI (C) demonstrated a histological response.
159SUMMARY OF DOSE CONSTRAINTS (TABLE 10.1)
TABLE 10.1 Dose Constraints for Brain Using SBRT
Brain Dose Guidelines |
|
Brain metastasis |
|
Lesion diameter ≤2 cm | 20–24 Gy |
Diameter >2 cm but ≤3 cm | 18 Gy |
Diameter >3 cm but ≤4 cm | 15–16 Gy |
Meningioma | 12–16 Gy (single fraction) or 20–25 Gy (four to five fractions) |
Pituitary adenoma | 22–24 Gy for secreting, ~15 Gy for nonsecreting (single fraction) or 20–24 Gy (five fractions) |
Acoustic neuroma | 12–13 Gy (single fraction) or 18 Gy (three fractions) |
Arteriovenous malformations |
|
<2 cm | 20 Gy (single fraction) |
2–3 cm | 18 Gy (single fraction) |
>3 cm | 15 Gy (single fraction) |
>3 cm | 20 Gy (five fractions) |
Vagal schwannoma/paragangliomas | 12–13 Gy (single fraction) or 20–25 Gy (four to five fractions) |
Trigeminal neuralgia | 80–90 Gy (single fraction to isocenter with 5-mm cone) |
Thalamotomy/pallidotomy | 130–140 Gy (single fraction to isocenter using 5-mm cone) |
Recurrent glioma | 15–24 Gy (single fraction based on size and location) |
Critical structuresa | Suggested dose limits |
Brainstem | V12 Gy <1 mL |
Optic apparatus | Maximum 8 or <12–14 Gy (three fractions) |
Brain | V12 Gy <5–10 mL |
Cochlea | Maximum 4.2 Gy (single fraction) or 6–8 Gy (three fractions) |
Plan evaluation |
|
Conformality index | Prescription isodose volume/target volume (1 is perfect, ~1.2 is good) |
Gradient index | Volume of 50% prescription isodose/volume of entire treatment isodose (~sharpness of dose falloff: want less than 3; can turn on 25% isodose line when placing shots) |
Heterogeneity index | Maximum dose/prescription dose (~hot spots) |
aFor several lesions in proximity, volume covered by 12-Gy isoline in normal brain tissue should be minimized.
SBRT, stereotactic body radiation therapy.
160REFERENCES
1. Timmerman R, Papiez L, McGarry R, et al. Extracranial stereotactic radioablation: results of phase I study in medically inoperable stage I non-small cell lung cancer. Chest. 2003;124:1946-1955. PubMed PMID: 14605072.
2. Nguyen T, Hsu W, Lim M, et al. Delivery of stereotactic radiosurgery: a cross-platform comparison. Neurol Res. 2011;33(8):787-791. doi: 10.1179/016164111X13123658647409
3. Brown Jr RD, Wiebers DO, Torner JC, et al. Frequency of intracranial hemorrhage as a presenting symptom and subtype analysis: a population-based study of intracranial vascular malformations in Olmsted Country, Minnesota. J Neurosurg. 1996;85:29-32. doi: 10.3171/jns.1996.85.1.0029
4. Crawford PM, West CR, Chadwick DW, et al. Arteriovenous malformations of the brain: natural history in unoperated patients. J Neurol Neurosurg Psychiatry. 1986;49:1-10. PubMed PMID: 3958721.
5. ApSimon HT, Reef H, Phadke RV, et al. A population-based study of brain arteriovenous malformation: long-term treatment outcomes. Stroke. 2002;33:2794-2800. PubMed PMID: 12468772.
6. Spetzler RF, Martin NA. A proposed grading system for arteriovenous malformations. J Neurosurg. 1986;65(4):476-483. doi: 10.3171/jns.1986.65.4.0476
7. Karlsson B, Lindquist C, Steiner L. Prediction of obliteration after gamma knife surgery for cerebral arteriovenous malformations. Neurosurgery. 1997;40:425-431. PubMed PMID: 9055280.
8. Friedman WA, Bova FJ, Mendenhall WM. Linear accelerator radiosurgery for arteriovenous malformations: the relationship of size to outcome. J Neurosurg. 1995;82:180-189. doi: 10.3171/jns.1995.82.2.0180
9. Flickinger JC, Pollock BE, Kondziolka D, et al. A dose-response analysis of arteriovenous malformation obliteration after radiosurgery. Int J Radiat Oncol Biol Phys. 1996; 36(4):873-879. PubMed PMID: 8960516.
10. Flickinger JC, Kondziolka D, Maitz AH, et al. Analysis of neurological sequelae from radiosurgery of arteriovenous malformations: how location affects outcome. Int J Radiat Oncol Biol Phys. 1998;40:273-278. PubMed PMID: 9457809.
11. Flickinger JC, Kondziolka D, Lunsford LD, et al. Development of a model to predict permanent symptomatic postradiosurgery injury for arteriovenous malformation patients. Arteriovenous Malformation Radiosurgery Study Group. Int J Radiat Oncol Biol Phys. 2000;46(5):1143-1148. PubMed PMID: 10725624.
12. Flickinger JC, Kondziolka D, Maitz AH, et al. An analysis of the dose-response for arteriovenous malformation radiosurgery and other factors affecting obliteration. Radiother Oncol. 2002;63:347-354. PubMed PMID: 12142099.
13. Colombo F, Pozza F, Chierego G, et al. Linear accelerator radiosurgery of cerebral arteriovenous malformations: an update. Neurosurgery. 1996;34:14-21. PubMed PMID: 8121550.
14. Maruyama K, Kawahara N, Shin M, et al. The risk of hemorrhage after radiosurgery for cerebral arteriovenous malformations. N Engl J Med. 2005;352(2):146-153. doi: 10.1056/NEJMoa040907
15. Lindquist C, Steiner L. Stereotactic radiosurgical treatment of malformations of the brain. In: Lundsford LD, (ed.). Modern Stereotactic Neurosurgery. Boston, MA: Martinus Nijhoff; 1988:491-505.
16. Schuknecht HF. Pathology of the Ear. Cambridge, MA: Harvard University Press; 1974:428.
17. Yamakami I, Uchino Y, Kobayashi E, et al. Conservative management, gamma-knife radiosurgery, and microsurgery for acoustic neurinomas: a systematic review of outcome and risk of three therapeutic options. Neurol Res. 2003;25: 682-690. doi: 10.1179/016164103101202075
18. Koos WT, Day JD, Matula C, et al. Neurotopographic considerations in the microsurgical treatment of small acoustic neurinomas. J Neurosurg. 1998;88(3):506-512. doi: 10.3171/jns.1998.88.3.0506
19. Karpinos M, Teh BS, Zeck O, et al. Treatment of acoustic neuroma: stereotactic radiosurgery vs. microsurgery. Int J Radiat Oncol Biol Phys. 2002;54(5):1410-1421. PubMed PMID: 12459364.
20. Brackmann DE. Middle cranial fossa approach. In: House WF, Luetje CM, (eds.). Acoustic Tumors. Vol 2. Baltimore, MD: University Park Press; 1979:15-41.
21. Bremond G, Garcin M, Magnan J. Preservation of hearing in the removal of acoustic neuroma (‘Minima’ posterior approach by retrosigmoidal route). J Laryngol Otol. 1980;94:1199-1204. PubMed PMID: 7430779.
22. Cohen NL, Ransohoff J. Preservation of hearing in acoustic neurinoma surgery. In: Samii M, Jannetta PJ, (eds.). The Cranial Nerves: Anatomy, Pathology, Pathophysiology, Diagnosis, Treatment. Berlin, Germany: Springer Verlag; 1981:561-568.
23. Sarmiento JM, Patel S, Mukherjee D, et al. Improving outcomes in patients with vestibular schwannomas: microsurgery versus radiosurgery. J Neurosurg Sci. 2013;57(1):23-44. PubMed PMID: 23584218.
24. Lunsford LD, Niranjan A, Flickinger JC, et al. Radiosurgery of vestibular schwannomas: summary of experience in 829 cases. J Neurosurg. 2013;119(suppl):195. PubMed PMID: 25077305.
25. Williams JA. Fractionated stereotactic radiotherapy for acoustic neuromas. Int J Radiat Oncol Biol Phys. 2002;54(2): 500-504. PubMed PMID: 12243828.
26. Norén G, Greitz D, Hirsch A, et al. Gamma knife surgery in acoustic tumours. Acta Neurochir Suppl (Wien). 1993;58: 104-107. PubMed PMID: 8109269.
27. Combs SE, Welzel T, Schulz-Ertner D, et al. Differences in clinical results after LINAC-based single-dose radiosurgery versus fractionated stereotactic radiotherapy for patients with vestibular schwannomas. Int J Radiat Oncol Biol Phys. 2010;76:193-200. doi: 10.1016/j.ijrobp.2009.01.064
28. Kondziolka D, Lunsford LD, McLaughlin MR, et al. Long-term outcomes after radiosurgery for acoustic neuromas. N Engl J Med. 1998;339:1426-1433. doi: 10.1056/NEJM199811123392003
29. Prasad D, Steiner M, Steiner L. Gamma surgery for vestibular schwannoma. J Neurosurg. 2000;92:745-759. doi: 10.3171/jns.2000.92.5.0745
30. Friedman WA, Bradshaw P, Myers A, et al. Linear accelerator radiosurgery for vestibular schwannomas. J Neurosurg. 2006;105(5):657-661. doi: 10.3171/jns.2006.105.5.657
31. Fukuoka S, Takanashi M, Hojyo A, et al. Gamma Knife radiosurgery for vestibular schwannomas. Prog Neurol Surg. 2009;22:45-62. doi: 10.1159/000163382
32. Régis J, Pellet W, Delsanti C, et al. Functional outcome after gamma knife surgery or microsurgery for vestibular schwannomas. J Neurosurg. 2002;97(5):1091-1100. doi: 10.3171/jns.2002.97.5.1091
33. Myrseth E, Møller P, Pedersen PH, et al. Vestibular schwannoma: surgery or gamma knife radiosurgery? A prospective, nonrandomized study. Neurosurgery. 2009;64(4):654-661. doi: 10.1227/01.NEU.0000340684.60443.55
34. Pollock BE, Driscoll CL, Foote RL, et al. Patient outcomes after vestibular schwannoma management: a prospective comparison of microsurgical resection and stereotactic radiosurgery. Neurosurgery. 2006;59:77-85. doi: 10.1227/01.NEU.0000219217.14930.14
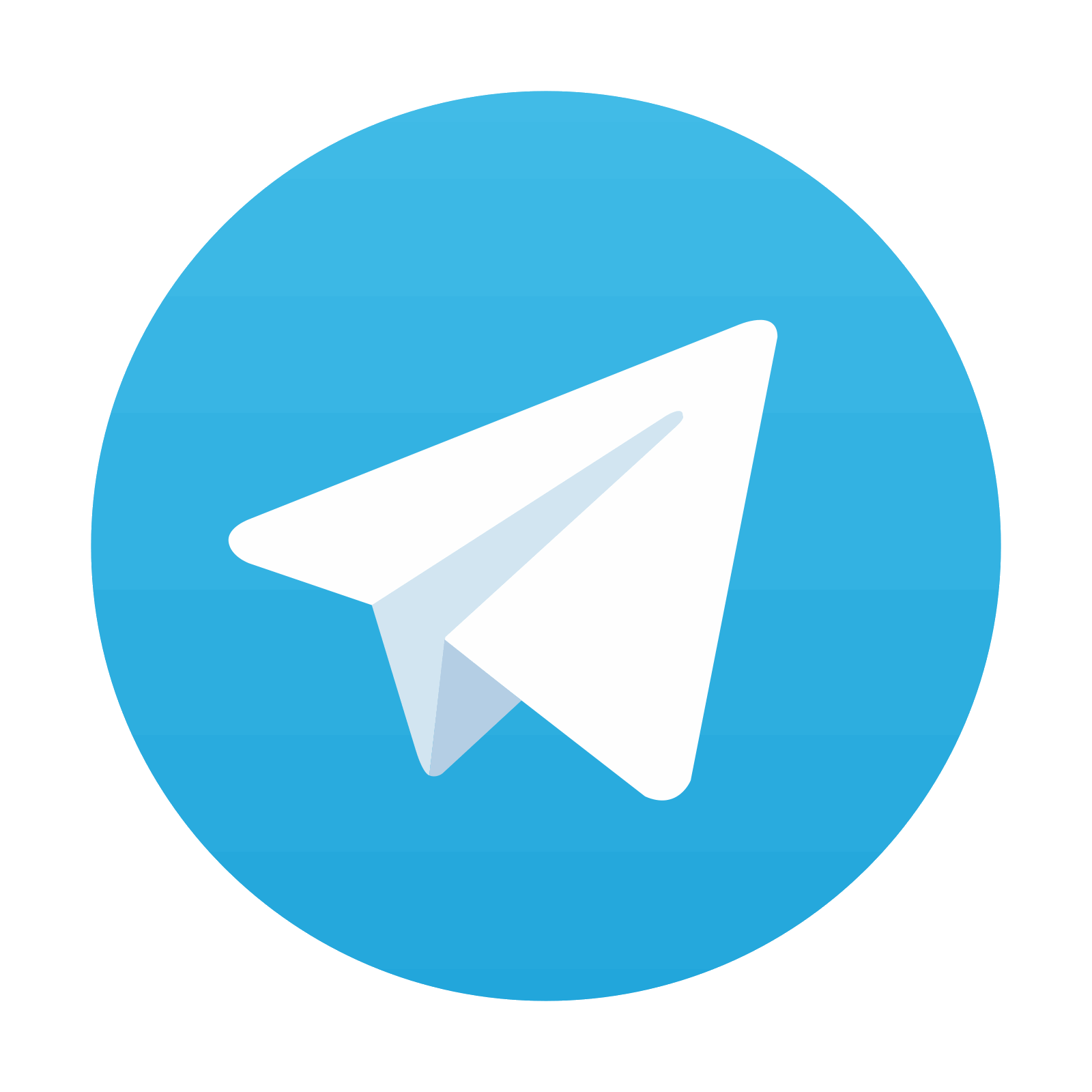
Stay updated, free articles. Join our Telegram channel
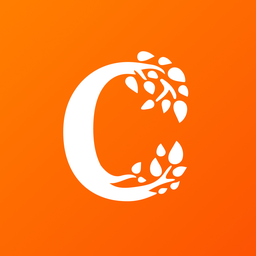
Full access? Get Clinical Tree
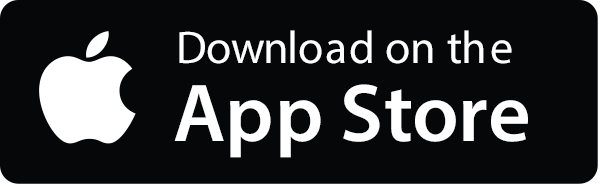
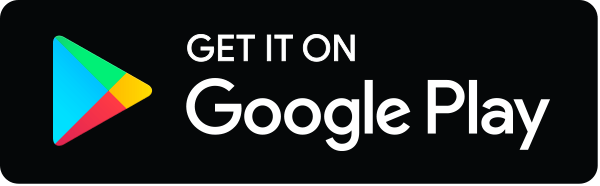