Functional magnetic resonance (fMR) imaging for neurosurgical planning has become the standard of care in centers where it is available. Although paradigms to measure eloquent cortices are not yet standardized, simple tasks elicit reliable maps for planning neurosurgical procedures. A patient-specific paradigm design will refine the usability of fMR imaging for prognostication and recovery of function. Certain pathologic conditions and technical issues limit the interpretation of fMR imaging maps in clinical use and should be considered carefully. However, fMR imaging for neurosurgical planning continues to provide insights into how the brain works and how it responds to pathologic insults.
Key points
- •
The use of functional magnetic resonance (fMR) imaging in the preoperative setting allows neurosurgeons to identify the location of patient-specific eloquent cortices adjacent to brain tumors with a high degree of certainty.
- •
fMR imaging helps neurosurgeons plan the operation and maximize the resection of the lesion while at the same time avoiding the adjacent eloquent cortex, thus, diminishing neurologic complications, including motor or speech function.
- •
Good patient performance is very important to achieve optimal fMR imaging results. Patients with neurologic deficits may need to have the paradigms modified.
- •
The blood oxygenation level–dependent fMR imaging method has several limitations that can make it difficult to acquire consistent and accurate data or to correctly interpret images. Knowledge of these pitfalls and artifacts will allow the radiologist to optimize the performance and interpretation of the fMR imaging scans.
Importance of functional magnetic resonance imaging
The main clinical application of functional magnetic resonance (fMR) imaging has been in preoperative brain tumor surgery planning. In brain surgery, it is essential to maximize the resection of the lesion (tumor or arteriovenous malformation) while at the same time avoiding the adjacent eloquent cortex. An eloquent cortex is defined as an area of gray matter essential for performing specific functions, such as sensation; movement; speech; vision; and higher cortical function, including memory.
Traditionally, before the advent of fMR imaging, the eloquent cortices were identified intraoperatively by physiologic methods, such as electrocorticography or somatosensory-evoked potentials. However, direct cortical stimulation has several drawbacks. First, this procedure requires a craniotomy and carries associated risks. Cortical mapping is restricted to the exposed surface of the brain; as a result, the cortex in the deep sulci cannot be adequately mapped. Also, cortical mapping occurs following a craniotomy, with the brain exposed, when patients are brought out of anesthesia. This mapping is known as awake mapping . In this setting, patients may have difficulty cooperating with task performance during cortical stimulation, especially when mapping higher function, such as language.
Performing preoperative fMR imaging, however, has several distinct advantages. First, preoperative fMR imaging does not require the use radioisotopes like positron emission tomography, which means that patients can be scanned multiple times without risk before the surgery. Second, using specific functional tasks decided by the location of the tumor, fMR imaging could generate cortical activity maps showing activated voxels adjacent to the tumor. With the meaningful functional maps, neurosurgeons can decide whether to perform a resection, biopsy, or not to operate at all. Thus, fMR imaging can help avoid an unnecessary craniotomy. Third, fMR imaging can elucidate whether the lesion is ipsilateral or contralateral to patients’ dominant language areas. If one can establish with a high degree of certainty that the lesion is contralateral to the dominant language areas, then the neurosurgeon can avoid awake mapping. Fourth, preoperative fMR imaging may influence the trajectory that a surgeon will take for resecting the tumor to bypass an important functional area and maximize tumor resection while minimizing damage to surrounding areas. However, it should be stressed that fMR imaging does not eliminate the need for intraoperative cortical mapping. If the tumor involves or is adjacent to the motor cortex or the language areas, the surgeon will most likely have to resort to cortical mapping to optimize the resection and avoid iatrogenic injury.
Importance of functional magnetic resonance imaging
The main clinical application of functional magnetic resonance (fMR) imaging has been in preoperative brain tumor surgery planning. In brain surgery, it is essential to maximize the resection of the lesion (tumor or arteriovenous malformation) while at the same time avoiding the adjacent eloquent cortex. An eloquent cortex is defined as an area of gray matter essential for performing specific functions, such as sensation; movement; speech; vision; and higher cortical function, including memory.
Traditionally, before the advent of fMR imaging, the eloquent cortices were identified intraoperatively by physiologic methods, such as electrocorticography or somatosensory-evoked potentials. However, direct cortical stimulation has several drawbacks. First, this procedure requires a craniotomy and carries associated risks. Cortical mapping is restricted to the exposed surface of the brain; as a result, the cortex in the deep sulci cannot be adequately mapped. Also, cortical mapping occurs following a craniotomy, with the brain exposed, when patients are brought out of anesthesia. This mapping is known as awake mapping . In this setting, patients may have difficulty cooperating with task performance during cortical stimulation, especially when mapping higher function, such as language.
Performing preoperative fMR imaging, however, has several distinct advantages. First, preoperative fMR imaging does not require the use radioisotopes like positron emission tomography, which means that patients can be scanned multiple times without risk before the surgery. Second, using specific functional tasks decided by the location of the tumor, fMR imaging could generate cortical activity maps showing activated voxels adjacent to the tumor. With the meaningful functional maps, neurosurgeons can decide whether to perform a resection, biopsy, or not to operate at all. Thus, fMR imaging can help avoid an unnecessary craniotomy. Third, fMR imaging can elucidate whether the lesion is ipsilateral or contralateral to patients’ dominant language areas. If one can establish with a high degree of certainty that the lesion is contralateral to the dominant language areas, then the neurosurgeon can avoid awake mapping. Fourth, preoperative fMR imaging may influence the trajectory that a surgeon will take for resecting the tumor to bypass an important functional area and maximize tumor resection while minimizing damage to surrounding areas. However, it should be stressed that fMR imaging does not eliminate the need for intraoperative cortical mapping. If the tumor involves or is adjacent to the motor cortex or the language areas, the surgeon will most likely have to resort to cortical mapping to optimize the resection and avoid iatrogenic injury.
Basic fMR imaging principles
Blood oxygenation level–dependent (BOLD) fMR imaging, discovered by Seiji Ogawa, is a brain-mapping technique using oxyhemoglobin and deoxyhemoglobin in the blood vessels as an endogenous contrast agent to generate functional activation maps. BOLD fMR imaging is based on the following principle: an increase in neuronal activity causes an increase in the local oxygen extraction from the blood because of an increase of the cerebral metabolic rate of oxygen. This increase leads to an increase in paramagnetic deoxyhemoglobin, which drops the signal intensity. However, after several seconds, neuronal activity also causes an increase in cerebral blood flow (CBF) and cerebral blood volume, which leads to an increase in the flow of oxygenated blood and a consequential increase in oxyhemoglobin. For yet unknown reasons, the amount of oxygenated blood that arrives to support the active neurons far exceeds the metabolic need. This overcompensation of oxyhemoglobin leads to a reduction in the ratio of deoxyhemoglobin to oxyhemoglobin, which is measureable and is the basis for the BOLD fMR imaging signal.
Neuroanatomy (functional areas)
Motor
The primary motor cortex (M1) is involved in performing movement and is located in the posterior portion of the frontal lobe in the precentral gyrus. The motor and sensory systems both have a topographic organization, which means that each portion of the body has a specific location on the cortex. The foot and leg are represented along the interhemispheric fissure, the hand lateral to that of the foot and leg, and the tongue and face at the most lateral level ( Fig. 1 ). The motor hand region of the precentral gyrus can be identified in the axial plane as a knoblike structure that is shaped like an upside down (or inverse) omega (Ω) ( Fig. 2 ).
The supplementary motor area (SMA) is involved in motor planning and organization. Its location is on the midline surface of each hemisphere anterior to the primary motor cortex leg representation. The SMA consists of 2 parts: the rostral (pre-SMA) and the caudal (SMA proper). The SMA proper, anatomically closer to primary motor and sensory areas, is involved in sensory, motor planning, and word articulation. The main functions of pre-SMA are cognitive tasks and language. Recently, Peck and colleagues showed that a central SMA between the rostral and caudal SMA can be resolved using simultaneous motor and language functional tasks.
The primary somatosensory cortex, the main sensory receptive area for the sense of touch, is located in the postcentral gyrus, a prominent structure in anterior aspect of the parietal lobe of the human brain. The organization of the sensory homunculus parallels the organization of the motor homunculus.
Language
In most right-handed individuals, language function is predominantly located in the left hemisphere. However, left-handed people are more likely to be codominant (language function seen in both hemispheres) or, in rare cases, right-hemisphere dominant.
Language can be subdivided into productive and receptive areas or frontal and temporoparietal areas ( Fig. 3 ).
The frontal (productive or Broca) language area is responsible for the expressive component of language and is located in the inferolateral portion of the left frontal lobe. The Broca area contains 2 main parts: pars opercularis and pars triangularis of the inferior frontal gyrus ( Fig. 4 ). However, these subdivisions are not particularly relevant in clinical practice because the inferior frontal operculum as a whole is traditionally considered eloquent. The pars triangularis is located in the anterior portion of Broca area. The pars opercularis is located in the posterior region of the Broca area. Damage to the Broca area can result in telegraphic, dysarthric, nonfluent speech; semantic and phonemic paraphasia; or even mutism.
The dominant receptive speech area (Wernicke area) resides mostly in the posterior superior temporal gyrus of the left hemisphere just posterior to the primary auditory gyrus. The Wernicke area is associated with the comprehension of language. Damage to this region may result in fluent aphasia; semantic, phonemic paraphasia; circumlocutions; and word-finding difficulty.
Secondary language areas include the middle frontal gyrus, the supplementary motor area, the insula, and the supramarginal and angular gyri. The middle frontal gyrus–premotor area, is a large area located just superior to the Broca area. Its function includes verbal working memory. Damage produces varied deficits ranging from nothing to dysarthria and anomia. The SMA is located in the superior frontal gyrus and is delimited posteriorly by the foot motor area. Damage to the anterior, language portion of the SMA causes a syndrome characterized by paucity of speech and occasionally mutism. However, patients often recover completely from damage to the language part of the SMA within weeks to months. The insula is located deep to the frontal operculum. It is likely involved in ventilatory needs during speech and end-stage speech production. Damage produces variable language deficits also ranging from nothing to word-finding difficulty and speech apraxia. The supramarginal and angular gyri are wrapped around the posterior portion of the sylvian fissure. The main functions of these areas include language perception, processing of auditory and visual input, and the comprehension of language. Damage to these areas usually results in alexia (inability to read) and agraphia (inability to write).
However, one must stress the great variability in language organization from one person to another. This point was recently emphasized in a careful study, conducted by Sanai and colleagues, using direct cortical stimulation. This variability of language organization further stresses the need for preoperative fMR imaging and intraoperative cortical stimulation.
Task and paradigm selection
Functional tasks are tasks specially designed for use with fMR imaging and are designed to increase neuronal activity in specific cortical areas. The functional tasks can be performed with one of 2 types of paradigms: block and event related. Each type of paradigm requires a resting and active state. The most common type in clinical fMR imaging is block-design paradigms. When performing a block paradigm, patients alternate between ON (active state) and OFF (resting state) periods of equal or unequal duration. Varying the duration of ON and OFF periods can be effective in decreasing noise from the scanner, heartbeat, and respiration. For example, to identify the hand motor area, a block paradigm might consist of alternating finger tapping and resting periods, each lasting 20 seconds. Usually, the paradigms repeat 5 to 6 times to increase power during statistical analysis.
In event-related paradigms, patients perform a single short event (usually, less than 4 seconds), such as swallowing or clenching a fist, followed by the rest period similar in duration to the block paradigms. This type of paradigm is used to investigate the neuronal or hemodynamic response to a specific single event. Event-related paradigms are particularly useful when an estimation of the hemodynamic response is desirable; but their limitations include long acquisition times, low statistical power, and corresponding complicated statistical analyses. Hence, this type of paradigm is not commonly used with clinical fMR imaging. Block designs are more effective at detecting an averaged fMR imaging signal because patients perform the same task over a period, for example, a 10- or 20-second period, and the design will increase the power to detect activated regions associated with a specific task. So, this type of detection can be advantageous in patients who may have impaired function or cognitive capacity.
General Motor Paradigms
Motor fMR imaging paradigms should be performed if there is a close anatomic relationship between the lesion and the motor homunculus, even if there are no motor symptoms. Commonly used paradigms to localize the motor strip include finger tapping, tongue motion, wiggling toes, or sensory foot or hand stimulation. In the finger-tapping task, patients are asked to sequentially tap their fingers avoiding arm or shoulder motion. Also, finger tapping can be changed to fist clenching or to sequential motion of the fingers from thumb to pinky finger, which can better capture premotor areas as well as the primary motor gyrus. However, in the authors’ experience in the brain tumor patient population, there is little practical difference in the exact type of hand-motor paradigm that is performed. The hand homunculus takes up a large portion of the motor cortex and gives a good motor signal, so hand paradigms should always be performed when localization of the motor strip is in question.
When performing tongue movement, patients are asked to keep their mouth closed and make a small sweeping tongue motion against the back of their teeth. Even small motion can elicit a strong fMR imaging signal; so large mouth movements should be avoided because they make it difficult to keep the patients’ head still during the fMR imaging scan.
The preservation of foot and leg mobility is essential in brain tumor surgery because loss of function will leave patients wheel-chair or bed bound. To complicate matters, the location of the foot motor homunculus is much more difficult from routine anatomic images. In addition, the foot motor homunculus is located deep in the interhemispheric sulcus making it inaccessible to direct cortical stimulation. As with other fMR imaging paradigms, it is very essential to keep the patients’ head still. Therefore, the foot-motion paradigms must be performed carefully with no ankle motion. Extensive ankle movement (performed concurrently with the paradigm) can lead to movement of the entire body, including the head, which will cause artifacts in the fMR imaging data that are very difficult to eliminate, even with sophisticated software.
Passive sensory paradigms, such as foot or hand stimulation by the examiner, can be helpful with patients who are paretic or elderly patients. Sensory paradigms will preferentially activate the postcentral (sensory) gyrus, from which the location of the corresponding motor homunculus can be extrapolated. However, these results should be interpreted with caution because fMR imaging activity usually activates both the sensory and motor gyri.
Language
The main goal of language fMR imaging is to lateralize and localize the brain area processing language function relative to the tumor. Tasks should be designed to target productive or frontal areas as well as receptive or temporoparietal areas.
Productive speech tasks are generally tasks that require patients to generate words in response to specific cues. These tasks are considered fluency tasks. During a phonemic fluency task, patients are given a letter and asked to generate words that begin with that letter. In the semantic fluency task, patients are asked to generate words that fit a given category, for example, fruits or vegetables. Another productive paradigm is verb generation whereby patients are given nouns and are asked to generate verbs. An example can be the word baby , to which patients will generate words such as cries , crawls , smiles , and so forth. Verb-generation tasks show more specificity than fluency tasks but have less variability in measuring hemispheric dominance.
Receptive speech tasks generally involve reading or listening to aurally presented words or reading a visually presented sentence and filling in the most appropriate answer. One more important receptive speech task requires patients to answer simple questions asked aurally, such as the following: What do you shave with? What color is grass? This type is generally known as auditory responsive naming .
It should be mentioned that despite tailoring the language paradigm to the lesion location, most language tasks will activate both frontal and posterior language areas to some degree because these areas are highly cooperative. However, it is more difficult to measure posterior language areas than frontal language areas.
An important question when performing language paradigms is whether they should be performed silent (covert) or vocalized (overt). Certain investigators recommend overt paradigms. The advantage of vocalized (overt) paradigms is that the investigator is able to confirm that patients are actually performing the required paradigm. Obviously, if patients are not performing the paradigm, the results of the fMR imaging will be useless. Additionally, the patients’ responses can be quantified, for example, how many of the questions did the patient answer correctly. However, for patients with a clinical brain tumor, the authors recommend silent (covert) paradigms. The authors have found that the main problem in patients with brain tumors is head motion, which often results from articulation of responses. In the authors’ practice, they use the real-time fMR imaging analysis to monitor patient responses and to ensure as much as possible that patients are performing the task correctly (see later discussion).
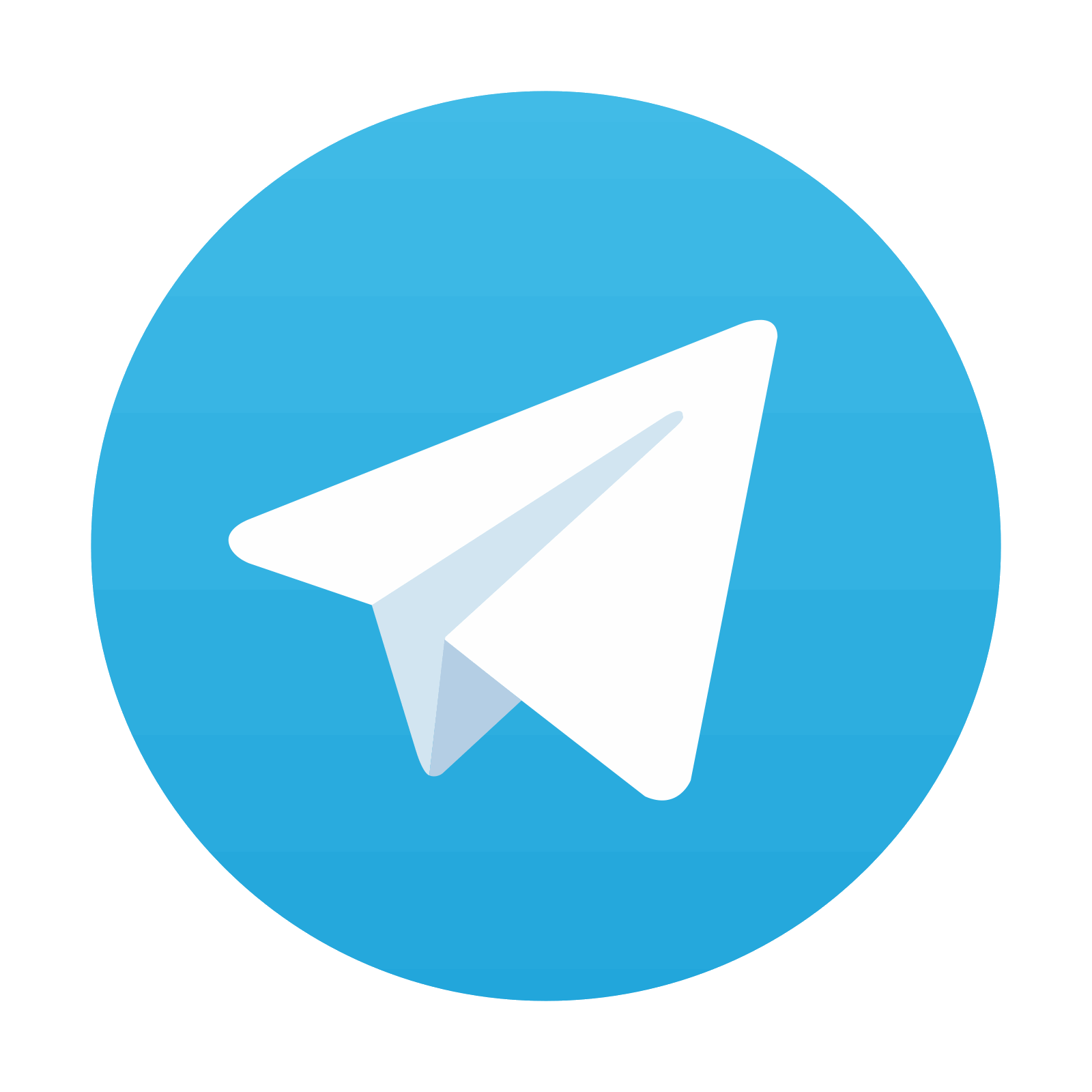
Stay updated, free articles. Join our Telegram channel
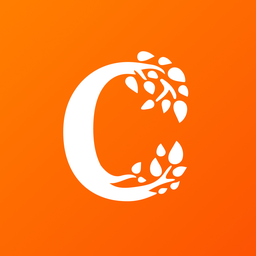
Full access? Get Clinical Tree
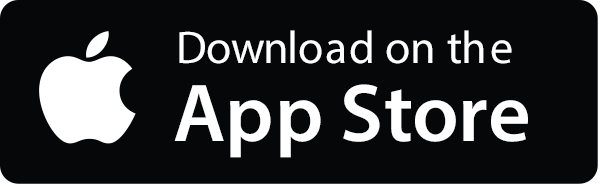
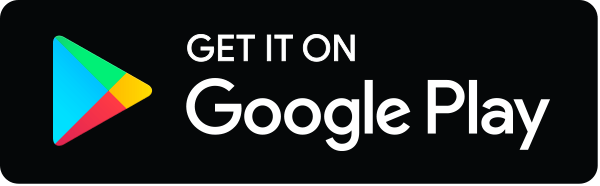