Magnetic resonance (MR) angiography is a powerful tool for the evaluation of cervical and intracranial vasculature. Both noncontrast and contrast-enhanced MR angiography can provide exquisite vascular contrast and detail without the use of ionizing radiation. More advanced techniques such as time-resolved MR angiography and parallel imaging provide dynamic information in rapid fashion. This article describes the basic principles and techniques of MR angiography image acquisition.
- •
Magnetic resonance (MR) angiography is a powerful tool for evaluation of cervical and intracranial vasculature.
- •
Both noncontrast and contrast-enhanced MR angiography can provide exquisite vascular contrast and detail without the use of ionizing radiation.
- •
At present, the performance of contrast-enhanced 4-dimensional MR angiography is comparable with that of computed tomographic angiography.
Introduction
Contrast-enhanced (CE) magnetic resonance (MR) angiography has emerged as a noninvasive, robust, high-resolution imaging technique for the evaluation of vascular disease. CE MR angiography has been successfully applied to every vascular territory of the body using varying imaging strategies at multiple centers across the globe. The rapid evolution and success of this technique is reflected by its widespread acceptance in current clinical radiologic practice. The technique continues to progress, having evolved from noncontrast flow imaging techniques to the present-day dynamic CE 3-dimensional (3D) MR angiography at 3 T with high spatial and temporal resolution.
Recent improvements in hardware such as newer radiofrequency coils, higher field strength at 3 T, and newer imaging techniques such as time-resolved MR angiography, parallel imaging methods, and keyhole imaging have made MR angiography a nearly equipotent rival to computed tomographic (CT) angiography, without the radiation hazards of the latter. Although catheter digital subtraction (DS) angiography still remains the gold standard for vascular imaging, it is expensive and the risk of arterial catheterization, iodinated contrast agents, and radiation hazard warrant safer imaging techniques.
As with all other MR imaging techniques, a thorough understanding of the underlying principles and diagnostic pitfalls of MR angiography is critical for optimal use of this tool. This article aims to acquaint the reader with basic principles of CE MR angiography and pertinent technical considerations, safety issues, and clinical applications in neuroradiology. The strengths and weaknesses of CE MR angiography in comparison with other noncontrast MR angiography techniques, and the recent technical innovations that continue to further enable the clinical utility of this robust imaging technique, are also discussed.
Principles and techniques
CE MR angiography is based on the T1 shortening effect of intravenous paramagnetic contrast medium. By shortening the T1 relaxation time of blood, these contrast media help to produce images in which contrast is based on differences in T1 relaxation between arterial blood, venous blood, and surrounding tissue. The amount of contrast injected is sufficient to reduce the T1 relaxation time of arterial blood below the T1 relaxation time of stationary tissue. As a result, arterial blood appears brighter than other tissues and venous blood. The shortening of T1 relaxation time of arterial blood during the first pass is transient. Acquisition of 3D images is timed properly to use this effect maximally. Suppression of the background signal is achieved by application of a 3D radiofrequency spoiled gradient sequence. This action also helps to decrease the T2 contrast of the background tissue, resulting in higher T1-weighted images.
CE MR angiography is much less dependent on blood inflow or phase-shift effects, in contrast to earlier MR angiography techniques such as time-of-flight (TOF) MR angiography and phase-contrast (PC) MR angiography. Therefore, this technique is less affected by motion and flow-related artifacts. The T1 shortening of blood by the paramagnetic contrast (gadolinium chelates) combined with less dependency on inflow of blood allows for imaging in the plane of vessels, thereby decreasing the number of image sections required to cover a long vessel. This process allows for faster scan times.
The proper performance of CE MR angiography involves thorough understanding of the complex interplay between the contrast dose, acquisition timing, and postprocessing methods. As illustrated by Maki and colleagues, for optimal CE MR angiography, patient-specific parameters, contrast dynamics, and pulse-sequence configuration should be considered. Patient-specific parameters such as breath-hold timing and contrast delay should be precisely ascertained. Contrast dynamics such as dose, contrast injection rate, and proper timing with respect to k-space acquisition should be determined. Finally, pulse sequence should be properly configured with proper selection of repetition time (TR), echo time (TE), flip angle, readout bandwidth, number of acquisitions, and so forth. These considerations are briefly discussed here.
Principles and techniques
CE MR angiography is based on the T1 shortening effect of intravenous paramagnetic contrast medium. By shortening the T1 relaxation time of blood, these contrast media help to produce images in which contrast is based on differences in T1 relaxation between arterial blood, venous blood, and surrounding tissue. The amount of contrast injected is sufficient to reduce the T1 relaxation time of arterial blood below the T1 relaxation time of stationary tissue. As a result, arterial blood appears brighter than other tissues and venous blood. The shortening of T1 relaxation time of arterial blood during the first pass is transient. Acquisition of 3D images is timed properly to use this effect maximally. Suppression of the background signal is achieved by application of a 3D radiofrequency spoiled gradient sequence. This action also helps to decrease the T2 contrast of the background tissue, resulting in higher T1-weighted images.
CE MR angiography is much less dependent on blood inflow or phase-shift effects, in contrast to earlier MR angiography techniques such as time-of-flight (TOF) MR angiography and phase-contrast (PC) MR angiography. Therefore, this technique is less affected by motion and flow-related artifacts. The T1 shortening of blood by the paramagnetic contrast (gadolinium chelates) combined with less dependency on inflow of blood allows for imaging in the plane of vessels, thereby decreasing the number of image sections required to cover a long vessel. This process allows for faster scan times.
The proper performance of CE MR angiography involves thorough understanding of the complex interplay between the contrast dose, acquisition timing, and postprocessing methods. As illustrated by Maki and colleagues, for optimal CE MR angiography, patient-specific parameters, contrast dynamics, and pulse-sequence configuration should be considered. Patient-specific parameters such as breath-hold timing and contrast delay should be precisely ascertained. Contrast dynamics such as dose, contrast injection rate, and proper timing with respect to k-space acquisition should be determined. Finally, pulse sequence should be properly configured with proper selection of repetition time (TR), echo time (TE), flip angle, readout bandwidth, number of acquisitions, and so forth. These considerations are briefly discussed here.
Patient-specific considerations
Certain patient-specific parameters need to be addressed for optimal imaging, and include ascertaining which vascular structures need to be visualized, preventing patient movement during acquisition, and accurately determining the contrast travel time from the site of venous injection to the vascular structure of interest. Predicting the duration of breath hold is also important, more so for abdominal and chest studies.
Timing of Injection and Acquisition: k-Space Considerations
Proper timing of the bolus injection and the image acquisition is vital, as the T1 shortening of the contrast is transient. It is also important to understand the concept of k space and its relevance in image-acquisition strategies. The appropriate scan delay between beginning of the injection and beginning of the scan should be ascertained, given by the following equation:
Contrast travel time can be ascertained by different strategies. “Best-guess” technique involves making an educated best guess based on injection site, vascular territory involved, and patient characteristics such as age and cardiac output. “Test bolus” involves injecting 1 to 2 mL of gadolinium before the actual scan at the same rate as that planned for the actual injection. The contrast travel time is then determined visually or by using region-of-interest analysis. This time can then be used to calculate scan delay for the actual study, as per the equation above. “Automated bolus detection” involves visually monitoring a vascular structure for arrival of contrast material followed by “triggering” of centric acquisition once contrast arrival is detected.
The k space
MR images are collected as spatial frequencies placed in a 2-dimensional (2D) array known as the k space. The Fourier transformation is then applied to these spatial frequencies to produce an image. Hence, k space represents an array of data representing the Fourier transformation of an object. It is a mathematical construct that facilitates the visualization of different MR imaging techniques. The center of k space represents low spatial frequency data that determine image contrast, while the periphery of k space represents high spatial frequency data that determine image detail. By proper timing of the contrast injection, it is possible to have the maximum arterial gadolinium concentration at the time of central k-space filling, thereby maximizing contrast-to-noise ratio and signal-to-noise ratio. For a given gadolinium dose, the injection strategy is a trade-off between a fast injection (shorter T1, more intravascular signal) and long injection (more uniform T1, fewer artifacts).
Phase encoding is used to spatially encode the data acquired. For 3D imaging, there are 2 phase-encoding directions and a single frequency direction, and all the data are collected before reconstructing individual images. There are different ways in which this is done. Linear or sequential phase encoding refers to acquiring k-space data sequentially, beginning at the periphery of the k space so that central k-space data are acquired at the midpoint of the scan ( Fig. 1 ). Centric phase encoding refers to acquiring central k-space data at the beginning of the scan. The image contrast in centric 3D data acquisition is determined by signals at the beginning of acquisition. Centric phase encoding is more suited for CE MR angiography, as it is less prone to incomplete breath-hold artifacts. Elliptical centric phase encoding involves concentrating the center of k space into a shorter period of time than with centric k-phase encoding. This process further improves suppression of venous signals, and reduces respiratory and motion artifacts. Another modification of this technique is recessed centric encoding, whereby absolute center of the k space is recessed a few seconds after initiating the scan, helping to avoid the ringing artifacts caused by data acquisition at the center of the k space as the contrast bolus arrives, as seen with elliptical centric phase–encoding methods.
Contrast Dose
The T1 shortening effect of gadolinium is proportional to its blood concentration according to the following equation:
where R1 is T1 relaxivity of gadolinium chelate and [Gd] is gadolinium concentration in the blood.
Of all background tissues, fat has the shortest T1 relaxation time (270 ms at 1.5 T). Therefore, the amount of contrast chosen should be sufficient to decrease the T1 of blood to less than 270 ms. This goal is usually achieved by 0.05 to 0.3 mmol/kg of intravenous gadolinium injected at 0.5 to 4 mL/s. With newer MR angiography techniques at 3 T, the contrast dose required to produce the same image contrast is much less than that needed with conventional CE MR angiography at 1.5 T. This method is discussed in further detail later.
Contrast Injection Rate
Contrast injection rate determines the arrival time of the contrast at the targeted vascular bed. The trade-off is between fast contrast injection to achieve maximal arterial signal and slower injection to minimize artifacts from rapid intravascular signal changes. A double-dose contrast (0.2 mmol/kg of Gd) with an injection rate of about 2 mL/s is considered a good compromise for routine CE MR angiography.
Selection of TR, TE, Readout Bandwidth, and Flip Angle
TR
The TR should be kept as short as possible (<4 ms) without increasing the bandwidth. Decreasing the TR results in shorter acquisition time, which allows for multiphase imaging or increased spatial resolution. However, the signal-to-noise ratio decreases as TR is shortened. This effect can be compensated by faster injection rate that concentrates the contrast bolus.
TE
TE should also be as short as possible. Decreasing the TE results in less dephasing of protons, which in turn decreases the artifactual loss of signal. However, decreasing TE also tends to decrease the signal-to-noise ratio by widening the readout bandwidth. The TE routinely used in clinical CE MR angiography is usually less than 2 ms.
Readout bandwidth
Adjusting the readout bandwidth can alter TR, TE, and signal-to-noise ratio. High readout bandwidths allow for shorter TR and TE at the expense of signal-to-noise ratio (see Fig. 1 ). The bandwidth used for clinical CE MR angiography is usually 32 or 64 kHz.
Flip angle
Flip angle in the range of 20° to 60° is acceptable. Low flip angles are more suited for lower contrast doses, slow injection rates, and imaging with very low TR. For higher contrast doses and imaging with higher TR, higher flip angles are better. Usually flip angles in the range of 30° to 45° are used in clinical CE MR angiography ( Figs. 2 and 3 ).
Noncontrast MR angiography techniques
Noncontrast MR angiography techniques have been routinely used for intracranial imaging since the advent of MR imaging. These techniques are still relied on widely at various centers around the world, especially for initial evaluation of intracranial and neck vessels. Their use is limited by long acquisition times and multiple flow-related artifacts. However, there has been a renewed interest in these techniques because of concerns over safety of gadolinium-based contrast media in patients at high risk for nephrogenic systemic fibrosis (NSF). These techniques are also used in cases where intravenous access is difficult, in pregnant patients, or when gadolinium is contraindicated. The major noncontrast MR angiography techniques are discussed here.
Time-of-Flight MR Angiography
3D TOF MR angiography is the most commonly used MR angiography technique, especially for imaging intracranial and neck vessels. TOF MR angiography relies on suppression of background signal by slice-selective radiofrequency excitation pulses so that in tissue planes with high flow velocity, the incoming blood that is free of the excitation pulse results in increased signal intensity. Selective arteriograms or venograms are possible by applying a presaturation pulse to eliminate signal from a specific direction. TOF MR angiography can be used in 2D or 3D format; the former is more suitable for imaging of veins and the latter for intracranial and neck arteries. 3D format allows for isotropic voxels, but the time of acquisition is long. Because of the long acquisition time, the use of 3D TOF MR angiography is largely limited to intracranial and head and neck vessels, where respiratory artifacts are minimal ( Fig. 4 ).
MOTSA (Multiple Overlapping Thin Slice Angiography) is a hybrid of sequential 2D MR angiography and single-slab 3D TOF MR angiography, which results in isotropic high-resolution images and allows for larger anatomic coverage. Each overlapping subvolume is acquired sequentially and is then fused with other subvolumes to form the complete 3D volume. This technique is especially useful for evaluation of large anatomic regions in high resolution. One of the major drawbacks of TOF methods, apart from the long acquisition times, is the artifactual loss of flow signals or pseudo-occlusion in regions of complex flow or flow reversal, such as in subclavian steal.
Phase-Contrast MR Angiography
PC MR angiography is a technique whereby contrast is generated by exploiting the inherent differences in transverse magnetization that occur between stationary and moving tissues, resulting in a phase shift. The phase shift is proportional to the flow velocity in the vessel. The velocity-induced phase shift can also be quantified. PC MR angiography uses a flow-encoding gradient along multiple planes to visualize flow. Owing to the need for multiple flow-encoding gradients, the acquisition time is higher than similar 3D TOF acquisitions. PC MR angiography also requires preselection of velocity-encoding factor (Venc), based on whether faster moving arterial blood or slower moving venous blood has to be imaged. If the Venc chosen is too low, velocity aliasing occurs, whereas if the Venc chosen is too high, the vascular contrast-to-noise ratio is low. However, the strength of PC MR angiography is in providing directional information and in quantitatively assessing flow. PC MR angiography is probably the most useful noncontrast MR angiography technique for imaging intracranial veins. For instance, the use of PC MR angiography is critical in cases when there is a subacute thrombus in the venous sinuses, as this can be incorrectly interpreted as blood using TOF MR angiography.
Steady-State Free-Procession MR Angiography
Steady-state free-procession MR angiography is a technique that has applications in evaluation of thoracic, abdominal, and cardiac vessels. The technique generates contrast that does not depend on flow; rather, the T2/T1 ratio of blood is exploited. Very short TR times (<3 ms) are used, so images can be generated in less than 1 second and breath holding is not required. However, the images are prone to spin dephasing and off-resonance artifacts. The technique has been used for the evaluation of coronary vessels, in breath-holding as well as free-breathing formats. It has also been used for the evaluation of carotid vessels.
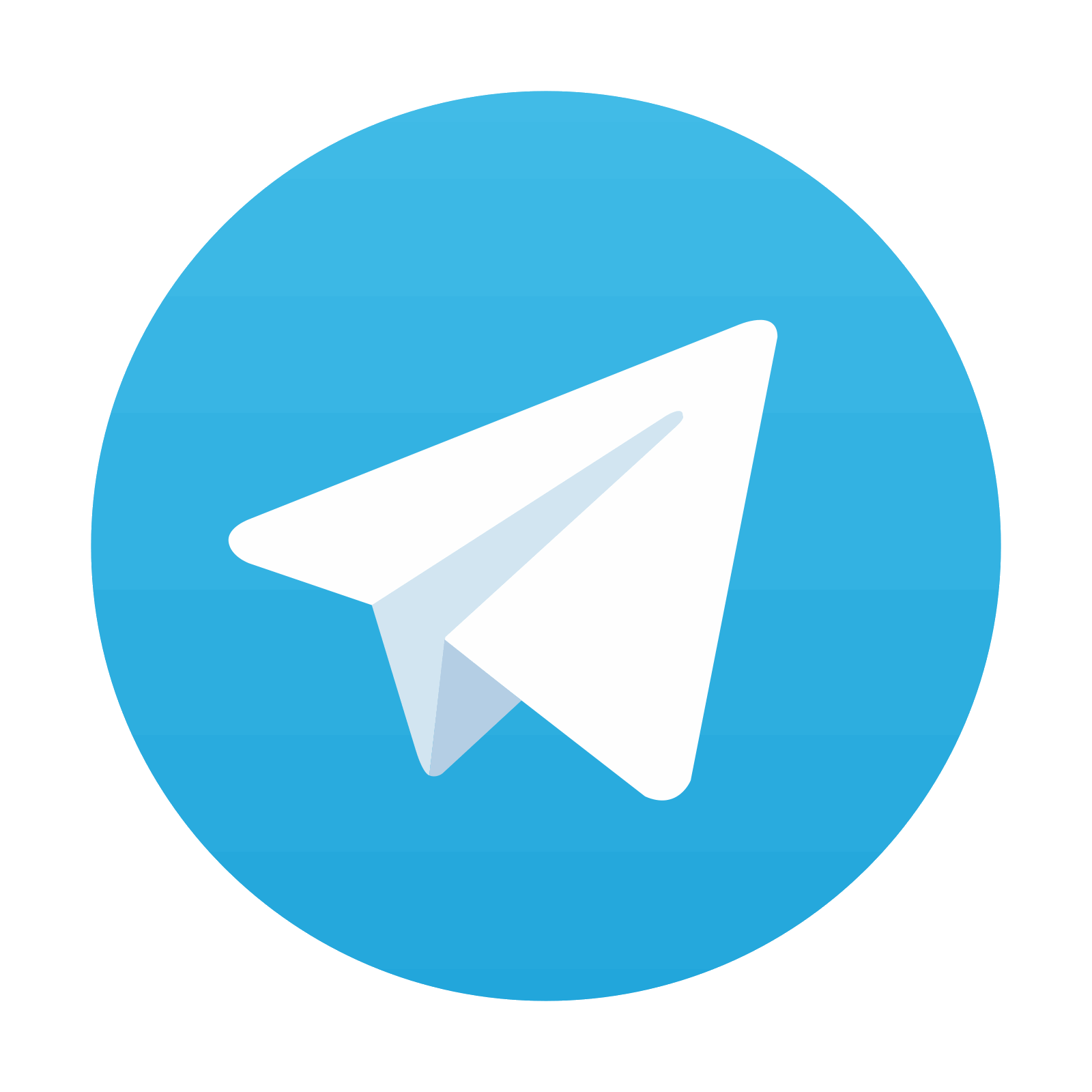
Stay updated, free articles. Join our Telegram channel
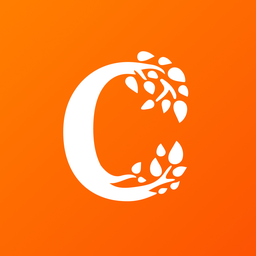
Full access? Get Clinical Tree
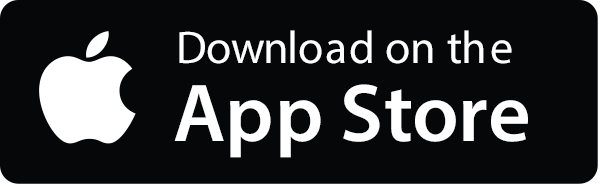
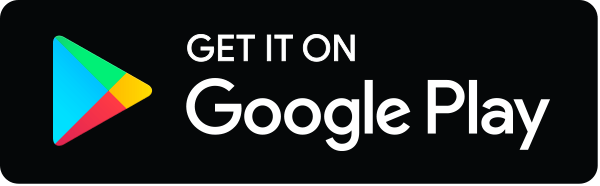