Coronary Artery Disease: Tissue Viability and Perfusion
Markus Schwaiger
Stephan G. Nekolla
Diagnosis of reversible left ventricular dysfunction in patients with heart failure remains an important clinical challenge. Single-photon emission computed tomography (SPECT) in combination with myocardial flow tracers plays an important role in the identification of reversible or irreversible perfusion defects as markers of tissue viability in segments with exercise included ischemia. Several positron emission tomography (PET) tracers of cardiac energy metabolism allow the semiquantitative or quantitative assessment of physiologic processes such as myocardial oxygen consumption, metabolic rate of glucose utilization, and myocardial blood flow. Metabolic imaging with PET offers a sophisticated means of assessing regional tissue viability in patients with advanced coronary artery disease (CAD) and impaired left ventricular function, as demonstrated in several clinical investigations. The assessment of relative and regional uptake covering the complete left ventricular volume with SPECT and PET represents an advantage over competing modalities. The classification of myocardial tissue into viable, hibernating, or scarred can be performed with high sensitivity, specificity, and reproducibility (see Fig. 30.8 and Table 30.2). The identification of hibernating and viable myocardium that will profit from revascularization (as proven by recovery of regional function) results in the high clinical utility of these tests. In addition, the combination of PET and SPECT with computed x-ray tomography (CT) allows the assessment of coronary anatomy and calcification, which, together with functional measurements by scintigraphic techniques, provides an important means of stratifying risk in patients with heart failure.
Introduction
The incidence of heart failure is increasing in industrialized nations, and the associated morbidity is consuming considerable national health care system resources. More than 50% of patients with impaired left ventricular function have coronary artery disease (CAD), as shown in large multicenter trials evaluating new drugs for the treatment of heart failure. It remains a diagnostic challenge to identify patients with significant CAD, because the extent and severity of ischemia as well as the degree of dysfunction determine the prognosis of patients with ischemic heart failure. Therefore, diagnostic tests are needed that not only address the quantification of left ventricular function and coronary anatomy but also provide indices of ischemic injury in this patient population. Because many of these patients had previous myocardial infarctions, the extent of remaining viable tissue is of clinical interest and also has been related to prognosis (1).
Several clinical studies have shown that myocardial dysfunction in patients with CAD may be reversible (2). Revascularization with either bypass grafting or percutaneous coronary intervention (PCI) may be beneficial in these patients in terms of relief of symptoms as well as
long-term survival. First results demonstrating the benefit of revascularization in patients with impaired left ventricular function used pharmacologic interventions in the catheter laboratory to demonstrate reversibility of regional wall motion before revascularization (2). Infusion of catecholamines identifies contractile reserve in the presence of resting myocardial dysfunction. Rahimtoola et al. introduced the term “hibernating myocardium” to refer to persistent left ventricular dysfunction in the presence of severe CAD that is reversible after revascularization (3). The pathophysiology underlying these phenomena is thought to reflect the down-regulation of function in the presence of reduced blood flow and oxygen supply (3,4). There is ongoing discussion of whether the left ventricular dysfunction is associated with the reduced myocardial resting blood flow or whether it represents repetitive ischemia in the presence of reduced coronary flow reserve (2). Independent of this academic discussion, the important clinical issue is the identification of reversibly injured myocardium. A number of noninvasive tests have emerged in recent years to address this issue. These tests for detecting viable myocardium can be divided into several groups: (a) assessment of contractile reserve during the infusion of catecholamine analogs or exercise by echocardiography or MRI; (b) the regional evaluation of perfusion and tracer retention using tracers such as technetium 99m [99mTc]sestamibi or thallium 201 (201Tl) in combination with SPECT; (c) the measurement of myocardial energy metabolism using PET, and (d) the identification of scar by delayed regional Gd-DTPA washout evaluated by MRI (late enhancement).
long-term survival. First results demonstrating the benefit of revascularization in patients with impaired left ventricular function used pharmacologic interventions in the catheter laboratory to demonstrate reversibility of regional wall motion before revascularization (2). Infusion of catecholamines identifies contractile reserve in the presence of resting myocardial dysfunction. Rahimtoola et al. introduced the term “hibernating myocardium” to refer to persistent left ventricular dysfunction in the presence of severe CAD that is reversible after revascularization (3). The pathophysiology underlying these phenomena is thought to reflect the down-regulation of function in the presence of reduced blood flow and oxygen supply (3,4). There is ongoing discussion of whether the left ventricular dysfunction is associated with the reduced myocardial resting blood flow or whether it represents repetitive ischemia in the presence of reduced coronary flow reserve (2). Independent of this academic discussion, the important clinical issue is the identification of reversibly injured myocardium. A number of noninvasive tests have emerged in recent years to address this issue. These tests for detecting viable myocardium can be divided into several groups: (a) assessment of contractile reserve during the infusion of catecholamine analogs or exercise by echocardiography or MRI; (b) the regional evaluation of perfusion and tracer retention using tracers such as technetium 99m [99mTc]sestamibi or thallium 201 (201Tl) in combination with SPECT; (c) the measurement of myocardial energy metabolism using PET, and (d) the identification of scar by delayed regional Gd-DTPA washout evaluated by MRI (late enhancement).
This chapter reviews the role of PET and SPECT in assessing regional tissue viability in patients with advanced CAD and impaired left ventricular function. Tracers of flow and cardiac energy metabolism are addressed, followed by a discussion of the available clinical results.
Table 30.1 Properties of Several Flow Tracers Used in the Assessment of Myocardial Perfusion | ||||||||||||||||||||||||||||||||||||||||||||||||||||||||||||||||||||||
---|---|---|---|---|---|---|---|---|---|---|---|---|---|---|---|---|---|---|---|---|---|---|---|---|---|---|---|---|---|---|---|---|---|---|---|---|---|---|---|---|---|---|---|---|---|---|---|---|---|---|---|---|---|---|---|---|---|---|---|---|---|---|---|---|---|---|---|---|---|---|
|
Flow Tracers
SPECT Flow Tracers
Several flow tracers have been introduced to assess myocardial perfusion under rest and stress conditions (Table 30.1). Potassium 43 (43K) and 201Tl were introduced because of their attractive physiological characteristics: high first-pass extraction, rapid blood clearance, and slow tissue washout (5). 201Tl became a very successful clinical flow tracer, but its low photon energy (80 to 90 KeV) and long physical half-life (73 hours) affect the image quality and limit the applicable dose with tolerable radiation exposure. Pohost et al. first described the use of 201Tl redistribution as marker of viable tissue (6). Based on the tissue kinetics of 201Tl, the myocardium can be characterized as normal (normal uptake, normal washout), ischemic (low uptake, delayed washout), or scar (low uptake, fast washout). Comparing early tracer retention at 15 to 30 minutes after injection with that of later timepoints (3 to 4 hours) allows for the separation of left ventricular segments with and without tracer redistribution. Some investigators proposed to monitor the redistribution process over prolonged time periods. Gutman et al. reported that in the presence of severe stenoses, defects that appeared fixed at 3 to 5 hours often displayed redistribution after 24 hours (7). Kiat et al. demonstrated subsequently that the redistribution pattern at 24 hours is more predictive for tissue recovery after revascularization than 3- to 4-hour data (8).
Although the presence of redistribution has been shown to serve as specific marker of viability, the absence of redistribution at 3 to 4 hours after tracer injection does not rule out tissue viability (8,9,10). In order to accelerate the redistribution of relative activity in the myocardium, a second 201Tl injection has been proposed (11). An injection of 201Tl at peak exercise with early imaging is followed by an injection
at rest and imaging after 3 to 4 hours. Based on this protocol, 201Tl scintigraphy has gained wide clinical acceptance as the most appropriate viability test using SPECT imaging.
at rest and imaging after 3 to 4 hours. Based on this protocol, 201Tl scintigraphy has gained wide clinical acceptance as the most appropriate viability test using SPECT imaging.
The 99mTc flow tracers have more suitable physical characteristics than 201Tl. A photon energy of 140 KeV and a half-life of 6 hours provide better count statistics because of less attenuation and higher allowable dose application. However, the first-pass extraction fraction of 99mTc[sestamibi] and [99mTc]tetrofosmin is lower than that of 201Tl (12,13,14). This may be a limitation for the assessment of perfusion at high flow rates but does not affect resting tracer distribution necessary for viability studies. Both 99mTc-labeled tracers are retained in viable myocardium for prolonged time periods. [99mTc]sestamibi is taken up in the mitochondria and retained by an electrochemical gradient. The relative distribution of both tracers at rest reflect perfusion and tissue viability (15).
PET Flow Tracers
Blood flow tracers can be classified based on their physiological behavior (see Table 30.1). Oxygen 15 [15O]water, for example, represents a freely diffusible tracer that washes in and out of myocardial tissue as a function of blood flow. The first-pass extraction of [15O]water in the heart is not diffusion limited, nor is [15O]water tissue extraction affected by any metabolic pathways (Fig. 30.1) (16,17,18). The second group of flow markers are radiotracers retained in myocardial tissue proportional to myocardial blood flow. For these radiopharmaceuticals, the initial tracer extraction (first-pass extraction) and their tissue retention are important factors defining their suitability as blood flow tracers. Nitrogen 13 (13N) ammonia is highly extracted by myocardial tissue in the form of [13N]ammonia (19,20,21). Within the tissue, the tracer can either back-diffuse into the vascular space or be trapped in the form of [13N]glutamine (Fig. 30.1). Ionic tracers such as rubidium 82 (82Rb), rubidium 81 (81Rb), and potassium 38 (38K) display tracer kinetics similar to those of 201Tl (12,22,23). Initial extraction of these compounds ranges from 50% to 70%. For both [13N]ammonia retention and ionic tracer extraction, a nonlinear relationship exists between blood flow and tissue tracer extraction (22,24). For methodological details of myocardial flow measurements by PET, please refer to an excellent recent review by Kaufmann and Camici (25).
Metabolic Tracers
Several PET radiopharmaceuticals have been developed to investigate the myocardial energy metabolism that depends on the oxidation of various substrates (Fig. 30.2). Under fasting conditions, the majority of cardiac adenosine triphosphate (ATP) production relies on the oxidation of free fatty acids (26,27).
Free fatty acids are avidly extracted by the myocardium where long-chain acyl-coenzyme A (CoA) is rapidly formed. Activated fatty acids are used in the synthesis of triglycerides or phospholipids. The majority of acyl-CoA, however, is transported via the carnitine shuttle into the mitochondria, where β-oxidation takes place. The end product of β-oxidation is acetyl-CoA, which enters the tricarboxylic acid (TCA) cycle, the final pathway of oxidative metabolism of all substrates. In the presence of high free fatty acid as well as low insulin plasma levels, only a small amount of glucose is extracted by the myocardium. However, in the postprandial state, glucose transport into the cell is enhanced, and the glycolytic rate increased. Even after carbohydrate loading, only about 30% to 50% of overall cardiac substrate metabolism depends on oxidative metabolism of glucose (28). During physical exercise, plasma lactate levels increase and contribute to myocardial energy metabolism (29).
Aside from the physiologic increase of glycolysis in the postprandial state, glycolysis plays an important role during and after myocardial ischemia. Experimental studies have shown that myocardial glucose transport and metabolism are up-regulated during myocardial ischemia with the production and release of lactate (30,31,32,33). However, after ischemic episodes, glycolysis remains enhanced, with evidence of oxidative and nonoxidative utilization of exogenous glucose (34,35). There is evidence that enhanced myocardial oxidative glucose metabolism persists after ischemic episodes (36,37). Biopsy studies in patients with severe CAD and chronic dysfunction of left ventricular segments have shown increased glycogen storage, evidence of
chronic alterations of glucose metabolism in hibernating myocardium (38). Such a metabolic pattern may reflect cell dedifferentiation with altered gene expression in repetitively ischemic myocardium with predominant glycolytic metabolism (39,40).
chronic alterations of glucose metabolism in hibernating myocardium (38). Such a metabolic pattern may reflect cell dedifferentiation with altered gene expression in repetitively ischemic myocardium with predominant glycolytic metabolism (39,40).
Fatty Acid Metabolism
The first radiopharmaceutical used in combination with PET for the assessment of regional cardiac metabolism was carbon 11 [11C]palmitate. [11C]palmitate is avidly taken up by the myocardium, with an extraction fraction of about 50%. The myocardial 11C activity clears in a biexponential pattern, including a rapid early and slow late phase. Experimental studies changing cardiac workload or cardiac substrate availability demonstrated changes in the relative contribution of the early and late phase of [11C]palmitate kinetics (41). Based on these studies, a relation was established between the early clearance phase and the relative contribution of β-oxidation to long-chain fatty acid metabolism (42,43). The contribution of the early clearance phase was correlated with myocardial oxygen consumption (41,43). Myocardial ischemia leads to impaired [11C]palmitate oxidation, as demonstrated by tracer kinetics (44,45). Experimental work correlating [11C]palmitate kinetics with ultrastructural tissue analysis in animals with ischemic injury revealed good correlation between lipid droplets and increased deposition of [11C]palmitate into a slow turnover pool (27). Quantification of mitochondrial function using [11C]palmitate is limited because of the influence of plasma substrate levels on the metabolic fate of exogenous fatty acids in terms of their incorporation into triglycerides or immediate oxidation. To avoid the complexity of substrate interaction defining the relative contribution of long-chain fatty acids and carbohydrates to overall oxidative metabolism, [11C]acetate has been proposed as an alternative probe to describe oxidative metabolism. [11C]acetate is converted to [11C]acetyl-CoA in the mitochondria and enters the TCA cycle. 11C activity equilibrates within TCA cycle intermediates, and 11C activity clears from myocardium in the form of [11C]CO2 (Fig. 30.3). Several studies have indicated that [11C]acetate kinetics, as assessed by dynamic PET imaging,
correlate closely with myocardial oxygen consumption, as assessed directly in the animal laboratory or by hemodynamic parameters in the clinical setting. Kinetics of [11C]acetate are only sparsely affected by substrate interactions and thus allow quantification of myocardial oxygen consumption (46,47,48,49,50). Tracer kinetic models have been introduced to quantitate [11C]acetate kinetics (51,52). However, most clinical studies use simple determination of clearance rates by using monoexponential curve fitting of regional myocardial 11C myocardial time–activity curves (47).
correlate closely with myocardial oxygen consumption, as assessed directly in the animal laboratory or by hemodynamic parameters in the clinical setting. Kinetics of [11C]acetate are only sparsely affected by substrate interactions and thus allow quantification of myocardial oxygen consumption (46,47,48,49,50). Tracer kinetic models have been introduced to quantitate [11C]acetate kinetics (51,52). However, most clinical studies use simple determination of clearance rates by using monoexponential curve fitting of regional myocardial 11C myocardial time–activity curves (47).
The use of [11C]palmitate and [11C]acetate in healthy volunteers indicates homogeneous uptake of these tracers within the normal left ventricular myocardium (47,53,54). The high initial extraction of [11C]palmitate and [11C]acetate allows the qualitative assessment of regional myocardial perfusion (41,55,56). The comparison of [11C]acetate uptake and [13N]ammonia blood flow measurements also indicates close correlation of results in patients with CAD, which points to the opportunity to assess flow and metabolism with a single tracer injection (see Fig. 30.3).
Glucose Metabolism
Fluorine 18 deoxyglucose (FDG) traces transmembranous transport as well as phosphorylation of exogenous glucose (57,58). FDG does not enter any further metabolic pathways but accumulates in myocardium proportional to glucose transport and phosphorylation (59,60). Very little dephosphorylation of FDG occurs in the myocardium. Experimental studies in isolated rabbit septum as well as canine models demonstrated a close relation of FDG uptake and exogenous glucose metabolism, measured by the Fick principle (60,61). However, FDG molecules display a different affinity for glucose transport and phosphor-ylation than does the glucose molecule itself (62). To correct for this discrepancy, a correction term is necessary for the quantification of exogenous glucose utilization of the myocardium by FDG. This correction term (lumped constant) is assumed to be constant under physiologic and most pathophysiologic conditions (63,64). Recent data, however, indicate that under rapidly changing conditions, the lumped constant may actually change as a function of altered affinities for glucose transport as well as for the hexokinase reaction (65,66). Exogenous glucose utilization can be quantified by using a simple fitting procedure of FDG myocardial kinetics and parametric display of regional metabolic data (67,68,69) (see Chapter 13).
The comparison of [11C]acetate uptake and clearance with regional FDG kinetics in normal volunteers demonstrated an inhomogeneity of regional glucose utilization in the heart (70). Regional FDG uptake is increased in the lateral wall of the left ventricle and slightly decreased in the area of intraventricular septum (71). Combined [11C]acetate and FDA myocardial substrate metabolism can be used for the quantification and determination of the relative contribution of glucose to overall oxidative metabolism (70,72). Such measurements have been performed to evaluate cardiac glucose metabolism in patients with insulin-dependent and non-insulin-dependent diabetes mellitus. In patients with a history of insulin-dependent diabetes, there was no significant difference in overall glucose utilization as compared with a control population if insulin was replaced by a euglycemic insulin clamp (73,74,75).
Clinical Application of SPECT Flow Tracers
201Thallium Imaging
There are numerous methods proposed to assess myocardial viability with 201Tl. The most commonly used imaging protocol with 201Tl includes stress injection of the tracer followed by reinjection and redistribution imaging 3 to 4 after tracer application. Bax et al. analyzed 33 studies with over 800 patients in a meta-analysis and found that 201Tl imaging using rest and stress protocols had a sensitivity of 86% and a specificity of 59% in predicting functional recovery following revascularization (76). The relatively low specificity may reflect the criteria used to classify viability as well as the protocol of data acquisition. Several investigators observed that the redistribution process following 201Tl injection may be prolonged in patients with severe coronary stenosis (7,8). Gutman et al. reported that in the presence of severe stenosis perfusion defects that appeared fixed at 3 to 6 hours after injection often showed redistribution at 24 hrs (7). Kiat et al. supported the observation that late imaging can be used to identify 201Tl redistribution in areas that were labeled fixed at 4 hours after injection (8). The investigation of Liu et al. following patients with single-vessel disease before and after coronary angioplasty confirm the notion that a fixed 201Tl defect does not exclude tissue viability (9). In this cohort of patients, 72% of patients with fixed defects also had evidence of myocardial viability after revascularization. This is further supported by studies comparing 201Tl kinetics with FDG-PET images. Brunken et al. demonstrated that patients who had fixed 201Tl defects displayed 18F-FDG uptake in the area of fixed defects (10). In this cohort, 58% of fixed 201Tl defects had evidence of viability based on metabolic imaging. Subsequently 201Tl reinjection was introduced to enhance the redistribution process. Dilsizian et al. reported that in patients with a fixed 201Tl defect on redistribution images, reinjection caused normalization of the defect in 49% of cases (77). Further studies by the same group indicated that 201Tl reinjection imaging has a much greater concordance with FDG-PET imaging than does conventional 201Tl redistribution, indicating a higher sensitivity for tissue viability by the reinjection procedure. In this study, 88% of viable segments by 201Tl reinjection protocols were also viable based on the FDG-PET studies (78).
Stress perfusion imaging may not be necessary in patients with known coronary artery disease who are scheduled for viability studies. Therefore, a number of investigators evaluated the value of rest-delayed 201Tl imaging as the preferred method for assessing regional tissue viability. In a group of 26 patients, Iskandrian found that 12 of 16 patients with normal or transient 201Tl defects showed improved ventricular function after surgery, whereas 2 out of 10 patients with fixed defects demonstrated some degree of functional recovery (79).
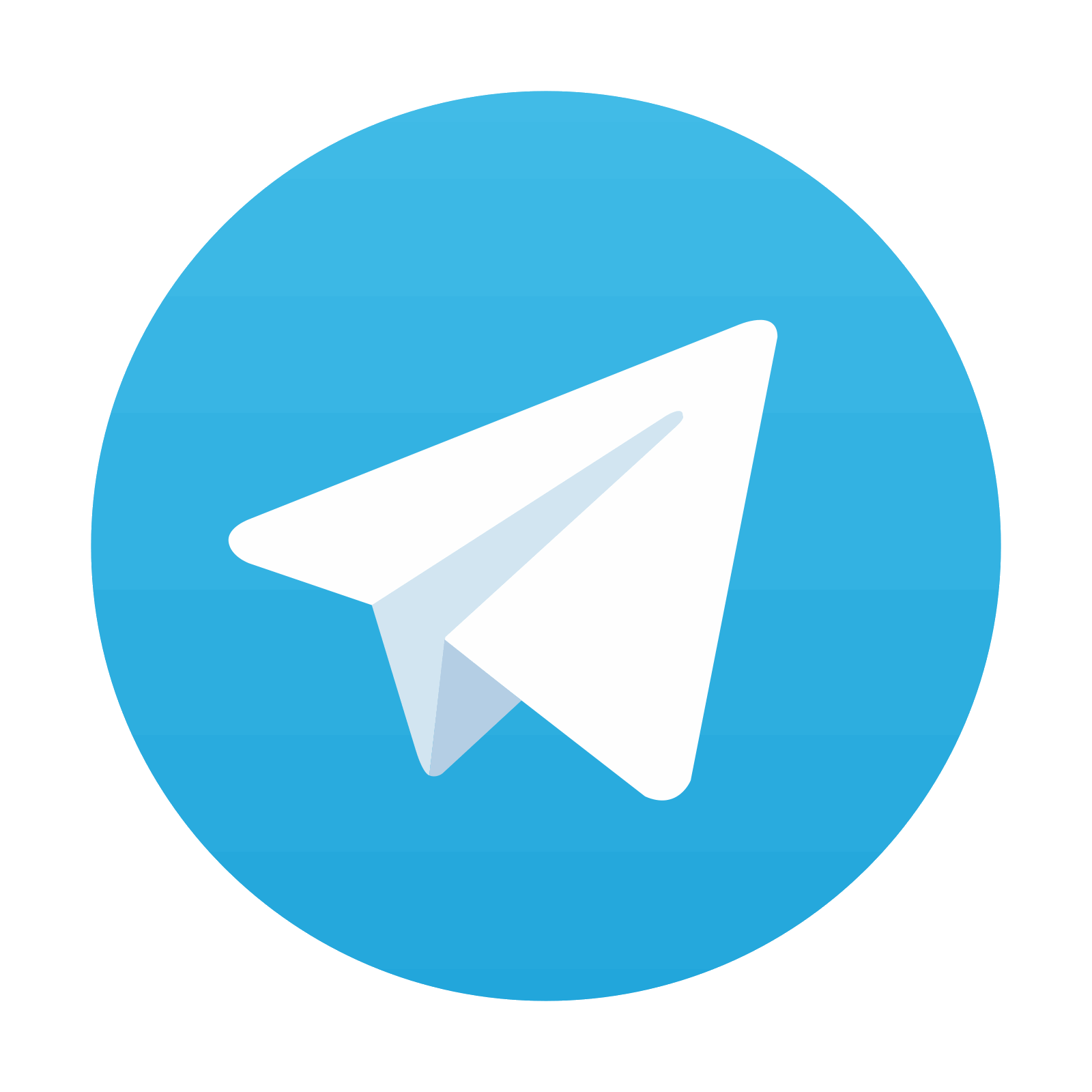
Stay updated, free articles. Join our Telegram channel
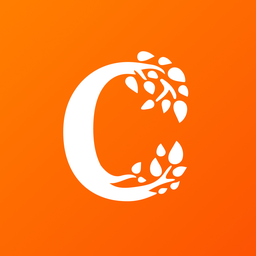
Full access? Get Clinical Tree
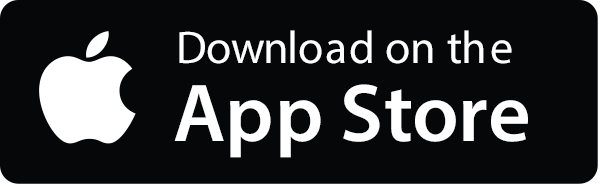
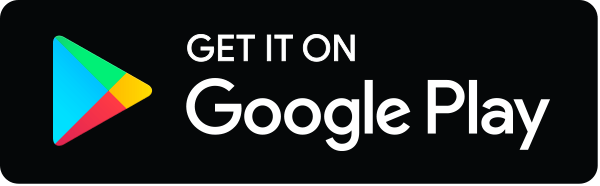