Traumatic Brain Injury
- Monique Anne Meyer
- Wayne S. Kubal
Traumatic brain injury (TBI) occurs when an external mechanical force results in tissue and cellular damage within the brain that may lead to permanent or temporary impairment of cognitive, physical, or psychosocial functions and a diminished or altered state of consciousness. There are two types of TBI: closed and penetrating. Closed TBI is an injury to the brain caused by movement of the brain within the skull. Causes may include falls, a motor vehicle crash, or being struck by or with an object. Penetrating TBI is an injury to the brain caused by a foreign object entering the skull. Causes may include firearm injuries or being struck with a sharp object. The simplest form of TBI is concussion, which may be defined as transient impairment of neurologic function with or without loss of consciousness occurring at the time of injury. About 75% of TBIs that occur each year are concussions or other forms of mild TBI.
TBI is one of the leading causes of disability and death in the Western world. According to the Centers for Disease Control and Prevention’s (CDC’s) report Traumatic Brain Injury in the United States: Emergency Department Visits, Hospitalizations, and Deaths, 2002-2006 , it has been estimated that at least 1.7 million people sustain a TBI in the United States per year. Of those individuals, about 52,000 die, 275,000 are hospitalized, and 1.365 million are treated and released from the emergency department (ED). The number of people with TBI who are not seen in a hospital or ED or who receive no care is unknown. TBI is a contributing factor to a third (30.5%) of all injury-related deaths in the United States.
The CDC data reveal that the leading causes of TBI are falls (35.2%), motor vehicle accidents (17.3%), collisions with moving or stationary objects (16.5%), and assaults (10%). Falls cause half (50%) of the TBIs among children ages 0 to 14 years and 61% of all TBIs among adults ages 65 years and older. Among all age-groups, motor vehicle crashes and traffic-related incidents were the second leading cause of TBI (17.3%) and resulted in the largest percentage of TBI-related deaths (31.8%). In every age-group, TBI rates are higher for males than for females with males ages 0 to 4 years having the highest rates of TBI-related ED visits, hospitalizations, and deaths.
TBI is heterogeneous in terms of pathophysiology, clinical presentation, and outcome, with case fatality rates ranging from less than 1% in mild TBI and up to 40% in severe TBI. A large proportion of patients with moderate to severe TBI are young adults; consequently, these patients often face decades of disability, with associated emotional, social, and financial difficulties. Approximately 5.3 million Americans are living with a TBI-related disability.
A severe TBI not only affects the life of an individual and his or her family, but it also has a large societal and economic toll. The estimated economic cost of TBI in 2010, including direct and indirect medical costs, is estimated to be approximately $76.5 billion. The cost of fatal TBIs and TBIs requiring hospitalization, many of which are severe, account for approximately 90% of the total of TBI medical care costs.
The mortality from TBI is related to the severity of brain injury as determined by the Glasgow Coma Scale (GCS). The GCS is used to provide a uniform approach to the clinical assessment of patients with acute head trauma and is the most frequently used means of grading the severity of injury within 48 hours. GCS is the sum of three components: eye opening, motor response, and verbal response. Persons with a GCS score of 3 to 8 are classified with a severe TBI, those with scores of 9 to 12 are classified with a moderate TBI, and those with scores of 13 to 15 are classified with a mild TBI ( Box 3-1 ).
The Glasgow Coma Scale (GCS) defines the severity of a traumatic brain injury (TBI) within 48 hours of injury.
Eye Opening
- ▪
Spontaneous = 4
- ▪
To speech = 3
- ▪
To painful stimulation = 2
- ▪
No response = 1
Motor Response
- ▪
Follows commands = 6
- ▪
Makes localizing movements to pain = 5
- ▪
Makes withdrawal movements to pain = 4
- ▪
Flexor (decorticate) posturing to pain = 3
- ▪
Extensor (decerebrate) posturing to pain = 2
- ▪
No response = 1
Verbal Response
- ▪
Oriented to person, place, and date = 5
- ▪
Converses but is disoriented = 4
- ▪
Says inappropriate words = 3
- ▪
Says incomprehensible sounds = 2
- ▪
No response = 1
The severity of TBI according to the GCS score (within 48 hours) is as follows:
- ▪
Severe TBI = 3-8
- ▪
Moderate TBI = 9-12
- ▪
Mild TBI = 13-15
Patients with TBI have also been classified into mild, moderate, and severe grades by the Brain Injury Interdisciplinary Special Interest Group of the American Congress of Rehabilitation Medicine based on a period of loss of consciousness, loss of memory for events immediately before or after the trauma, changes in mental status, and focal neurologic deficits. Mild head injury is defined as a traumatically induced physiologic disruption of brain function manifested by one of the following: any period of loss of consciousness; any loss of memory for events immediately before or after the accident; any alteration in mental state at the time of the accident (e.g., feeling dazed, disoriented, confused); or focal neurologic deficits, which may or may not be transient. However, the severity of the injury does not exceed the following: loss of consciousness of 30 minutes; after 30 minutes, an initial GCS score of 13 to 15; and posttraumatic amnesia not greater than 24 hours. Additional criteria for defining mild TBI include GCS score greater than 12; no abnormalities on computed tomography (CT) scan; no operative lesions; and length of hospital stay less than 48 hours. Moderate TBI is defined as length of stay at least 48 hours, GCS score of 9 to 12, operative intracranial lesion, and abnormal CT findings. Severe TBI is usually self-evident at the time of presentation.
Imaging Modalities
The goals of emergency imaging of TBI are to allow life-threatening injuries to be rapidly diagnosed and treated, to explain the findings on neurologic examination, and to establish a prognosis for the patient.
Computed Tomography
Noncontrast CT is the first imaging test performed in the ED setting for the evaluation of head trauma. The goal of emergency imaging is to depict lesions that need emergency neurosurgical treatment or that may alter therapy. When trauma is evaluated with CT, images are reviewed in brain, subdural, and bone settings at the workstation. Wide window settings aid in separating high-density blood from the high density of bone. Bone windows aid in the evaluation of fractures. Coronal and sagittal reformatted images should be provided to increase accuracy of interpretation and decrease misses.
CT perfusion allows the noninvasive evaluation of tissue perfusion. CT perfusion is performed by continuous scanning of a single slice or contiguous slices after the administration of iodinated contrast. The passage of the contrast bolus through the brain is analyzed to measure the time to peak density, the mean transit time, the cerebral blood volume, and the cerebral blood flow. CT perfusion can provide prognostic information about patients with severe TBI but is not widely used in the setting of acute TBI.
Magnetic Resonance Imaging
Magnetic resonance imaging (MRI) is reserved for showing lesions that could explain clinical symptoms and signs that are not explained by prior CT or to help define abnormalities seen on CT. Due to its superior contrast resolution for soft tissues, MRI is far more sensitive than CT in detecting all the different stages of hemorrhage, diffuse axonal injury (DAI), and ischemia. Standard sequences such as T1 weighted, T2 weighted, and fluid-attenuated inversion recovery (FLAIR) are usually performed as part of the examination. Detecting acute subarachnoid hemorrhage (SAH) is difficult if FLAIR imaging is not performed. The absence of beam-hardening artifact with MRI enables better visualization of the inferior frontal and temporal lobes and posterior fossa.
Gradient-recalled echo (GRE) sequences are especially sensitive to multiple blood breakdown products and are capable of depicting areas of microhemorrhage not well depicted on CT. Susceptibility-weighted imaging (SWI) is a high-spatial-resolution three-dimensional (3-D) gradient-echo magnetic resonance (MR) technique involving phase postprocessing that accentuates the paramagnetic properties of blood products such as deoxyhemoglobin, intracellular methemoglobin, and hemosiderin. When this technique is used, a mask is created to enhance the phase differences between susceptibility artifacts and surrounding tissues. The contrast-to-noise ratio is optimized by multiplying the mask and magnitude images. A minimal-intensity projection is created to facilitate differentiation between veins and focal lesion. The phase images are sensitive for depiction of regions of local alteration of the magnetic field (i.e., susceptibility) caused by various substances, such as hemorrhage and iron and other metals. SWI is three to six times more sensitive than conventional GRE sequences for the detection of small hemorrhages in DAI. A potential pitfall in SWI interpretation is that the signal intensity of veins depends on blood oxygenation. Veins are hypointense compared to arteries. The magnetic properties of intravascular deoxygenated hemoglobin and the resultant phase differences with adjacent tissues cause signal loss. To prevent misregistration of vessel versus focal lesions, the thickness of the minimal-intensity projection should be adjusted to the brain size to minimize partial volume effects, particularly in neonates.
Perfusion MRI methods exploit signal intensity changes that occur with the passage of a gadolinium-based contrast agent. As the contrast agent passes in high concentration through the microvasculature, susceptibility-induced T2 relation occurs in the surrounding tissues, which is seen as a decrease in signal intensity. The decrease in signal intensity is assumed to be linearly related to the concentration of gadolinium-based contrast agent in the microvasculature. The changes in the signal intensity of the tissues are quantitatively related to the local concentration of gadolinium-based contrast agent and are converted into a curve of concentration versus time. By applying tracer kinetics to the concentration-time curve of the first passage of the bolus of contrast agent, relative cerebral blood volume and cerebral blood flow maps are calculated.
The use of diffusion-weighted imaging (DWI) and diffusion tensor imaging (DTI) in assessment of patients with TBI is widely gaining acceptance. DWI is based on the random motion of water molecules, also known as brownian motion . This motion is affected by the intrinsic speed of water displacement depending upon the tissue properties and type (i.e., gray matter, white matter, cerebral spinal fluid [CSF]). DWI can detect changes in the rate of microscopic water motion. Water molecules within the ventricles diffuse freely, whereas water molecules within the cells have their diffusion restricted. Water molecules within the extracellular space have an intermediate diffusion. When there is movement of water from the extracellular space to the intracellular space, the overall diffusion within that volume of tissue becomes more restricted. This restricted diffusion correlates with cytotoxic edema and allows for the early detection of ischemic changes.
Apparent diffusion coefficient (ADC) is a measure of diffusion. This measure has been used as an indicator of edema. Recently it has been shown that the use of mean ADC in the whole brain is the best predictor of outcome among all degrees of TBI.
DTI incorporates pulsed magnetic field gradients into a standard MRI sequence that characterizes the local diffusivity (the magnitude of diffusion) of water. The fiber structure of the central nervous system can be evaluated using DTI by observing restricted water diffusion. In healthy white matter there is greater diffusion along the long axis of the axonal bundles than along the radial axis because of hindrance from the myelin sheath. Two key measures associated with water diffusion are diffusivity and anisotropy (the direction of water diffusion). DTI is based on the fact that microscopic water diffusion in white matter tracts tends to occur in one direction rather than randomly, which is termed anisotropy . The degree of anisotropy in a white matter region can be viewed as a reflection of the degree of structural integrity of white matter. A number of different measures of anisotropy can be used; one of the more commonly used is fractional anisotropy. Fractional anisotropy is sensitive to changes in white matter integrity and provides pertinent information regarding the degree of white matter damage, including factors such as myelin sheath thickness and axonal membrane integrity. Given that white matter damage is a predominant feature in TBI, there is a resultant decrease in fractional anisotropy values in severe TBI.
MR spectroscopy (MRS) allows the chemical environment of the brain to be examined noninvasively. The metabolites detected by MRS are sensitive to hypoxia, energy dysfunction, neuronal injury, membrane turnover, and inflammation. MRS localization techniques can be divided into single voxel and multivoxels. The most common brain metabolites that are measured with proton ( 1 H) MRS include N -acetyl aspartate (NAA), creatine (Cr), choline (Cho), glutamate, lactate, and myoinositol. Water-suppressed localized proton MRS of the brain can be performed at long or short echo times. At long echo times the human brain reveals four major resonances: a peak at 3.2 ppm, arising mainly from tetramethylamines, especially choline-containing phospholipids; a peak at 3.0 ppm, arising from Cr, either alone or as phosphocreatine; a peak at 2.0 ppm, arising from N -acetyl groups, especially NAA; and a doublet peak at 1.3 ppm arising from the methyl resonance of lactate, which is normally barely visible above baseline. NAA, an amino acid synthesized in mitochondria, is a neuronal and axonal marker that decreases with neuronal loss or dysfunction. Cr is a marker for intact brain energy metabolism. Cho is a marker for membrane synthesis or repair, inflammation, or demyelination. Lactate accumulates as a result of anaerobic glycolysis and, in the setting of TBI, may be a response to the release of glutamate. Signals from important compounds such as myoinositol (3.56 ppm) and glutamate (2.1 to 2.4 ppm) are preferentially obtained with short echo time measurements. Specifically, glutamate and immediately formed glutamine are excitatory amino acid neurotransmitters released to the extracellular space after brain injury and play a major role in neuronal cell death. Myo -inositol, an organic osmolyte located in astrocytes, increases as a result of glial proliferation. When interpreting MRS, comparisons are made with the Cr peak because it tends to be the most stable in a variety of conditions. The normal NAA peak is nearly twice the height of the Cr peak, whereas the normal Cho peak is only slightly smaller than the Cr peak ( Fig. 3-1 ).
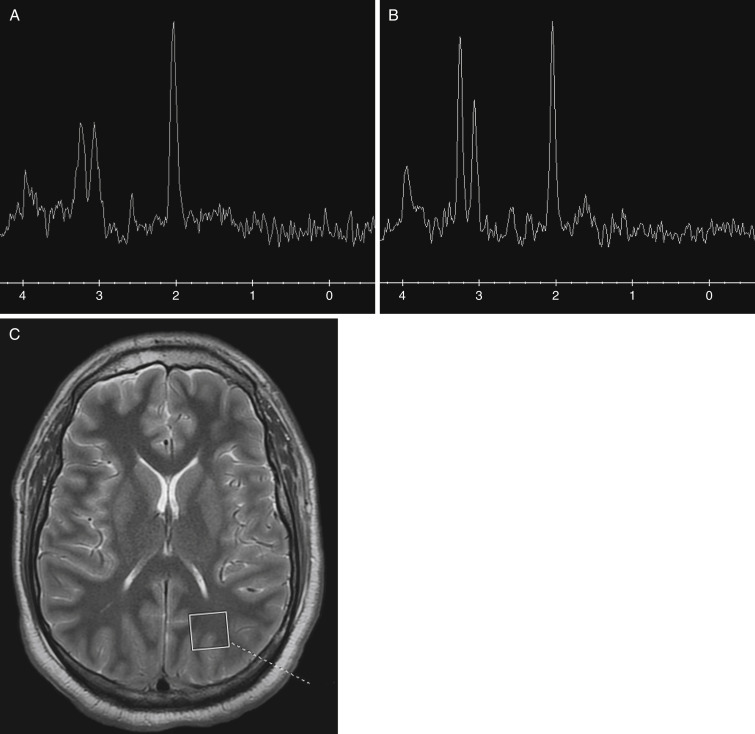
The expression of lactate in the brain is usually an expression of a pathologic condition. These include impairment of the oxidative metabolism or infiltration of macrophages and inflammatory cells. All these conditions take place after TBI. Studies have consistently found large increases in Cho level and diffuse decreases in NAA level in the brains of TBI patients. The diffuse elevation of Cho level after TBI may reflect membrane disruption, increased cell membrane turnover, and/or astrocytosis. The findings of widespread low levels of NAA in TBI should be interpreted as due to permanent neuro-axonal damage or in acute conditions such as TBI, might also depend on potentially reversible axonal dysfunction to postinjury mitochondrial impairment (see Fig. 3-1 ). Reduced NAA (or NAA/Cr, NAA/Cho) level is apparent within the first 24 hours of injury, possibly as early as 8 hours after TBI.
Classification of Injury
Traumatic brain injuries may be separated into two major categories: primary injury and secondary complications. Primary injury and its primary complications are directly related to immediate impact damage, whereas secondary complications result from the primary injury over time. Primary injuries are considered irreversible, whereas secondary injuries are potentially preventable with efficient triage and stabilization.
Primary Injury
The primary brain injury is the physical damage to the parenchyma (tissue, vessels) that occurs during a traumatic event, resulting in shearing and compression of the surrounding brain tissue. Two different mechanisms are principally responsible for primary injury: (1) direct contact between the skull and an object and (2) inertial injury resulting from the differential accelerations between white and gray matter.
Secondary Complications
The secondary brain injury is the result of a complex process, following and complicating the primary brain injury in the ensuing hours and days. Secondary complications are temporally removed from the original trauma and result in brain injury, including increased intracranial pressure (ICP), hypoxia, infection, infarction, and herniation. Numerous secondary brain insults, both intracranial and extracranial or systemic, may complicate the primarily injured brain and result in secondary complications. Secondary, systemic brain insults are mainly ischemic in nature. In recent years, increased recognition, ICP and cerebral perfusion pressure (CPP) monitoring, and early respiratory and circulatory support have decreased the extent of secondary complications and improved outcomes among TBI patients. In addition, implementation of and adherence to guidelines advocating a structured and systemic approach to TBI patients have also improved outcomes.
Scalp and Skull Injury
Close examination of the scalp soft tissues should be one of the first steps in interpreting a trauma head CT. Identification of soft tissue injury helps the radiologist to pinpoint the site of impact, or the “coup” site. This site should be carefully inspected for the presence of an underlying skull fracture, as well as evidence of soft tissue laceration and foreign bodies.
Fractures of the skull and of the skull base can lead to several complications, including CSF leakage, neurovascular damage, meningitis, facial palsy, deafness, carotid dissection, and carotid cavernous fistula. Fractures can also serve as an indication of adjacent hematomas and can indicate the possibility of associated delayed complication, even if no initial hematoma is present. CT, including coronal and sagittal reconstructions, is the best method for the detection of skull fractures.
Extra-Axial Injuries
We will discuss four types of extra-axial fluid collections: epidural hematoma (EDH), subdural hematoma (SDH), subdural hygroma, and subarachnoid hemorrhage (SAH). Pneumocephalus, intracranial air, can occur in any of these four locations, as well as in the brain parenchyma, referred to as a pneumatocele .
The imaging appearance of posttraumatic fluid collections is best understood in relation to the meningeal layers covering the brain. The three meningeal layers are the dura mater, arachnoid mater, and pia ( Fig. 3-2 ). The pia is the deepest layer, covering the brain surface and lining the cortical gyri. It also lines the perivascular space, the Virchow-Robin space, which communicates with the subarachnoid space. This space normally contains a small amount of CSF. The arachnoid is superficial to the pia and is attached to the pia via innumerable arachnoid trabeculations. The subarachnoid space is the space between the arachnoid and pia.
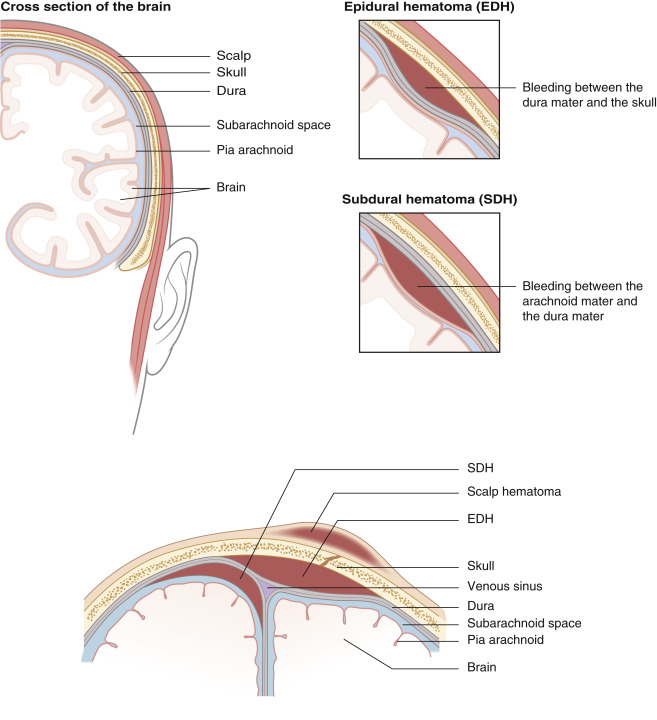
The subdural space lies between the inner layer of the dura and the arachnoid. Bridging cortical veins transverse the subdural space. Because the dura is firmly attached to the skull and the arachnoid is attached to the cerebrum, most brain motion occurs across the subdural space, placing the bridging cortical veins at risk for tear.
The dura is composed of two layers: an outer periosteal layer and an inner meningeal layer that forms the dural reflections such as falx cerebri and tentorium cerebelli. The periosteal and meningeal layers are tightly bound to each other and to the skull. The dura surrounds and supports the venous sinuses. The two leaves of the dura separate only to enclose the venous sinuses. The potential space between the periosteal layer of the dura and the inner table of the skull is the epidural space. Running within the epidural space are branches of the middle meningeal artery. The periosteal layer is tightly adherent to the margins of the cranial sutures; therefore epidural fluid collections do not cross the suture margins.
Epidural Hematoma
EDHs are caused by contact forces that are of high magnitude and short duration. The potential space between the inner table of the skull and the dura is the epidural space. At the moment of impact the dura may be stripped away from the inner table, and extravasated blood from injured meningeal vessels, diploic veins, or dural sinuses fill the newly created space. EDHs are more common in adolescents because the dura is not as firmly attached to the skull as it is in older people. EDHs are usually biconvex in configuration because of firm adherence of the dura to the inner table and its attachment to the sutures. The most common cause of EDH is head trauma with skull fracture (adjacent skull fracture is present in greater than 90% of cases) in the temporoparietal region crossing the vascular territory of the middle meningeal artery. Arterial EDHs are far more common than venous EDHs in the supratentorial region. Venous EDH is most often noted in the pediatric population and carries a lower incidence of skull fracture. In addition, skull fracture may not be present in children due to the increased plasticity of the calvaria. Venous EDHs that are associated with skull fractures typically occur infratentorially from injury to the transverse sinus or sigmoid sinuses. Venous EDHs are associated with a better prognosis, as they rarely increase in size because the pressure exerted by extravasated venous hemorrhage is insufficient to cause further stripping of the dura from the skull. Venous EDHs often involve a basilar fracture extending to the torcular Herophili or the transverse sinus. These hematomas are uncommon (15%) as compared with arterial EDHs (85%).
Symptoms after injury are directly related to the mass effect from the EDH. The “classic” presentation is a lucid interval as the lesion expands before causing midline shift and deterioration; however, fewer than 20% of the patients demonstrate this presentation. Expanding high-volume EDHs can produce a midline shift and subfalcine herniation of the brain. Compressed cerebral tissue can also impinge on the third cranial nerve, resulting in ipsilateral pupillary dilation with contralateral hemiparesis or extensor motor response.
EDHs are not limited by the falx and tentorium. The CT appearance of both arterial and venous EDHs is that of a high attenuation, biconvex, extra-axial collection. Low attenuation regions within the collection may represent areas of active bleeding within the EDH. CT findings that are associated with an adverse clinical outcome in patients with an EDH include pterional location of the skull fracture, EDH thickness greater than 1.5 cm, EDH volume greater than 30 mL, location within the lateral aspect of the middle cranial fossa, associated midline shift greater than 5 mm, and the presence of active bleeding within the EDH. Because of their high density, acute EDHs are usually well seen in CT. MRI can also show these collections; however, it is typically less sensitive for the identification of the associated skull fractures. MRI is useful for evaluation of associated injuries that may be present.
Chronic EDHs reveal low density and peripheral enhancement, and they may be concave. Chronic EDHs should be distinguished from other epidural masses such as infection, inflammation, or tumor and from subdural lesions such as empyema.
Small EDHs may be managed conservatively. Acute EDHs isolated to the anterior aspect of the middle cranial fossa constitute a subgroup of traumatic EDHs with a benign natural history. It is postulated that they arise from venous bleeding because disruption of the sphenoparietal sinus. Emergency surgical decompression is nearly always needed for larger EDHs, hematomas associated with active bleeding, and hematomas associated with substantial mass effect. The surgical goal is to alleviate mass effect from the EDH before secondary injury occurs.
Subdural Hematoma
Hemorrhage in the potential space between the pia-arachnoid and the dura is termed a subdural hematoma . Hemorrhage may originate from cerebral veins, venous sinuses, or adjacent cerebral contusions. Acute SDHs are typically the result of significant head injury and are caused by the shearing of bridging veins. SDH can be seen in both the coup and contrecoup locations, with the contrecoup location being more common. The most common locations are along the cerebral convexities, the falx cerebri, and tentorium cerebelli. SDHs may cross suture lines but not dural reflections, such as the falx and tentorium. Older adults and infants are particularly susceptible to this injury because their bridging veins are stretched due to age-associated cerebral and cerebellar volume loss. Because of the loose connection between the arachnoid and the dura, an SDH spreads over a considerable area and usually has a crescent configuration overlying the convexity of the brain.
Small SDHs may be missed because of high-convexity locations, beam-hardening artifacts, or narrow window settings. A wider window (width, 200; level 70) helps to differentiate acute hemorrhage from bone. MRI is more sensitive than CT in the detection of small SDHs and tentorial or interhemispheric SDHs. A potential pitfall is an ossified cerebral falx, which contains bone marrow with a high T1 signal, which may mimic an SDH on MRI.
SDHs may be classified as simple or complicated. Simple SDHs are without associated brain injury, and complicated SDHs occur with parenchymal injury. Lesions occurring at the time of injury are acute, 3 days to 3 weeks are subacute, and greater than 3 weeks are chronic. Acutely, these collections usually have a uniform high density on CT, although approximately 40% are heterogeneous in density. Causes of low-density regions within an acute SDH include unclotted blood in an early stage of hematoma development, serum extruded into the early phase of clot retraction, and CSF within the subdural space because of an arachnoid tear. Occasionally the acute SDH may be isodense or hypodense due to underlying anemia. As the SDH becomes subacute to chronic, the collection becomes isodense to brain in the subacute phase and hypodense to brain in the chronic phase ( Fig. 3-3 ). During the subacute period an isodense phase can occur, and the collection can be difficult to detect on noncontrast CT but more conspicuous on MRI ( Fig. 3-4 ). MRI can be useful in indicating the chronicity of the SDH by showing blood in different oxidation states. Imaging clues to the presence of an isodense SDH are abnormally thick cortex, white matter buckling, and the presence of mass effect.
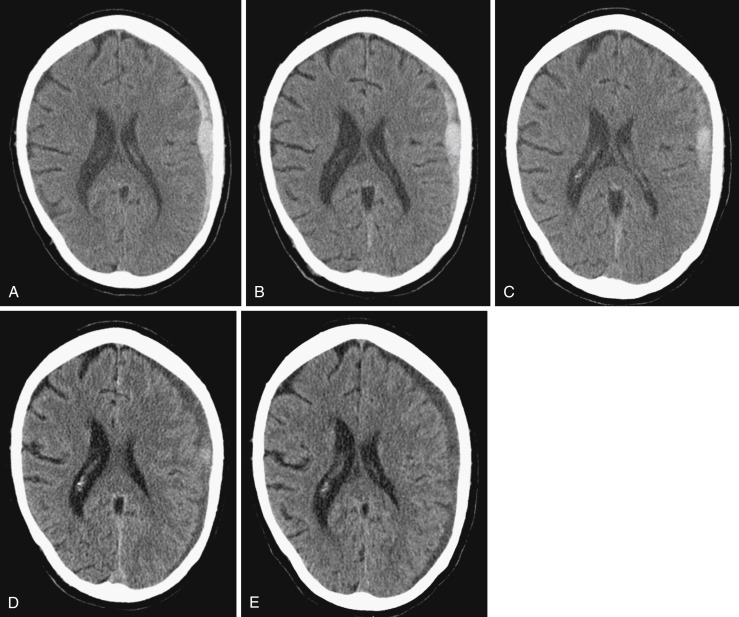
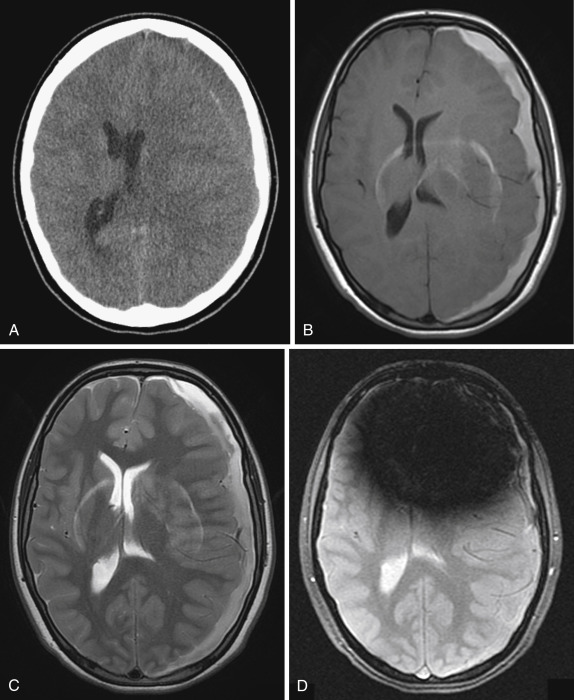
By CT, chronic SDHs may not be distinguishable from subdural hygromas ( Fig. 3-5 ). However, the walls of hygromas do not enhance. Subacute to chronic subdural lesions enhance because of vascularization of the subdural membranes, which are formed between 1 and 3 weeks after injury. Repeated episodic bleeding within an SDH results in fibrous septations and compartments within the hematoma ( Fig. 3-6 ). Subacute and chronic hematomas may also display layering with dependent cells and cellular debris and an acellular supernatant.
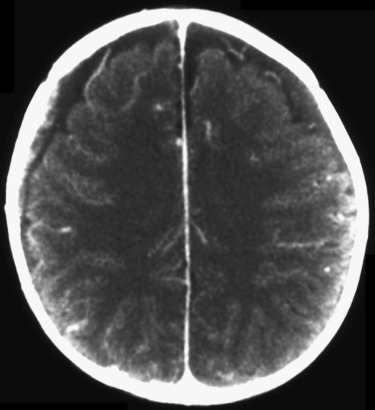
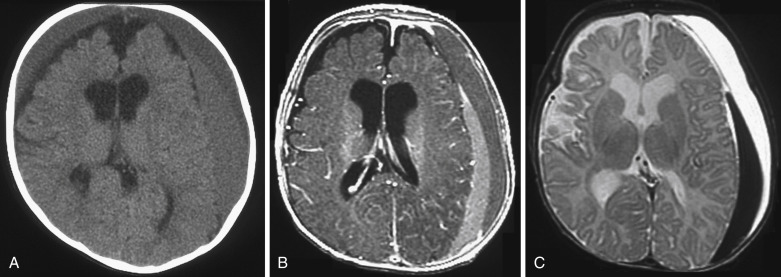
The prognosis for patients with SDH is variable and is related to the extent of the primary underlying brain injury rather than the SDH itself.
Subdural Hygroma
Collections of fluid within the subdural space that have imaging characteristics similar to CSF are termed hygromas . They can result from trauma and can occur acutely as a tear in the arachnoid membrane with CSF collecting in the subdural space or result from the chronic degeneration of an SDH.
In subdural hygroma the collection is between the dura and the arachnoid, forcing the arachnoid inward along with the cortical veins. In patients with volume loss the cortical veins on the surface of the brain are seen extending across and within the fluid collection. With MRI one might see high protein, which reveals itself as high signal intensity on FLAIR and small amounts of residual hemorrhage on gradient echo imaging.
Subarachnoid Hemorrhage
Traumatic SAH is the result of injury to small, bridging cortical vessels on the pia or arachnoidal leptomeninges that cross the subarachnoid space. Hemorrhage from an intracerebral hematoma may decompress directly into the subarachnoid space or dissect into the ventricular system. The most frequent location of traumatic SAH is the convexity, followed by the fissures and basal cistern ( Fig. 3-7 ). SAH may induce cerebral vasospasm, which is more likely to occur when SAH is accompanied by SDH, intraventricular hemorrhage, cerebral contusion, or intracerebral hemorrhage. Aneurysmal SAH can typically be differentiated from traumatic SAH based on the distribution of the hemorrhage. Aneurysmal SAH tends to be distributed centrally, commonly involving the suprasellar, interpeduncular, and prepontine cisterns, whereas posttraumatic SAH has a peripheral distribution.
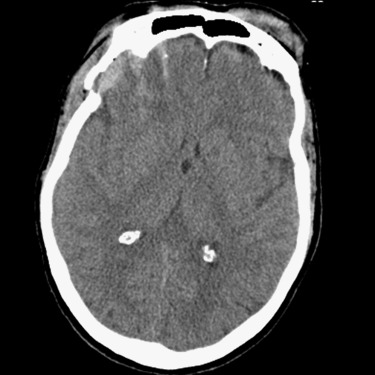
On CT, acute SAH presents as high attenuation within the subarachnoid space. It is important to differentiate true SAH from pseudo-SAH present in diffuse cerebral edema. In diffuse cerebral edema the elevated ICP causes an increase in the density of the subarachnoid space (pseudo-SAH), which may be the result of decreased CSF in the subarachnoid space or engorgement and dilatation of the small pial vessel in the subarachnoid space. With MRI, FLAIR images are needed to detect small areas of acute or subacute SAH. A pitfall of the FLAIR sequence is that it does not differentiate between abnormalities that appear increased in signal intensity such as hemorrhage, inflammatory exudate, neoplastic leptomeningeal infiltration, vascular slow flow, and hyperoxygenation during sedation or anesthesia. Subacute and chronic SAH are difficult to detect on CT because SAH is typically isodense to CSF on CT. Subacute and chronic SAH is best detected on MRI. Subacute hemorrhage is best detected with FLAIR imaging with altered signal intensity within the CSF, and chronic blood products are best detected on GRE and SWI with weighted images appearing as areas of decreased signal intensity.
Communicating hydrocephalus may result as a chronic sequela of prior SAH. As SAH is cleared from the CSF, the blood is phagocytized and transferred to the arachnoid villi. The arachnoid villi become distended and filled with phagocytes. They may become obliterated by the proliferation of connective tissue (“scarring”), causing impaired absorption of CSF.
Intra-Axial Injuries
Contusions
Contusion is bruising of the brain caused by direct contact and occurs when the brain surface rubs across the rough inner table of the calvaria. Capillary injury and edema are the result. The most common locations for brain contusions are the anterior and inferior surfaces of the frontal lobes as well as the temporal and occipital lobes. The differential acceleration of the brain relative to the calvaria in the setting of trauma results in abrasion and impaction of the brain along the inner table. Both coup and contrecoup injuries can be associated with intra-axial hemorrhage.
Most cerebral contusions are subtle on initial CT scanning but tend to become larger and more obvious during the first 3 days post trauma because of progression of edema and hemorrhage ( Fig. 3-8 ). Nonhemorrhagic contusions are best seen acutely with MRI on DWI. Many superficial contusions initially are hemorrhagic, reflecting the rich vascular supply of the cortex. On T2-weighted MRI, an acute contusion has a central dark signal representing deoxyhemoglobin with surrounding hyperintensity representing edema. As the contusion becomes subacute, it appears hyperintense on T1, representing evolution of the blood products to methemoglobin with increased surrounding vasogenic edema. Subacute contusions and hematoma can mimic brain abscess, infarct, or neoplasm if history of trauma is unknown, as it often demonstrates ring enhancement following intravenous contrast administration. Chronic contusion may present itself as an area of encephalomalacia or area of hemosiderin best seen on GRE and SWI sequences because they are of decreased signal intensity ( Fig. 3-9 ).
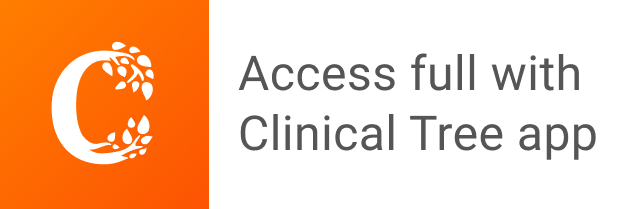