Fetal IVIM MRI
Until the mid-1970s, obstetricians could “image” the anatomy and pathophysiology of the living fetus by relying on their senses: manual palpation and auscultation were common practice apart from the rather exceptional cases where the use of conventional radiography or invasive methods was justified. The emergence of clinically feasible ultrasonography (US) complemented the diagnostic armamentarium with a method that directly visualized the developing fetus in vivo, in utero. US remains an indispensable and ubiquitous part of prenatal care and management, whereas the technically more complicated, time-consuming, and more expensive magnetic resonance imaging (MRI) has established itself as a valuable second line of investigation, especially for the evaluation of intracranial structures. Undoubtedly, the greatest advantages of fetal MRI are a larger field of view, independence of fetal position, and excellent tissue contrast, allowing detailed assessment of different aspects of human anatomy and pathology. We start this chapter with a short introduction to fetal MRI. MRI safety and the challenges and possible solutions of image acquisition and postprocessing options are discussed with particular attention. We then review the surprisingly few studies that have utilized the IVIM technique for prenatal diagnostics to date, concentrating on feasibility and reproducibility. By reviewing the literature regarding fetal motion correction studies, we outline a possible framework for acquiring and analyzing clinically usable fetal intravoxel incoherent motion (IVIM) MRI. We illustrate potential pathologies where IVIM MRI could be useful and discuss their relevance in the context of postnatal follow-up.
17.1 Fetal Magnetic Resonance Imaging
Today, fetal MRI is used in specialized diagnostic centers, and clinical research using fetal MRI has gained increasing attention since the 2000s. The most important enabling factor of this technique is perhaps the possibility to radically shorten imaging time, as for example by using single-shot fast spin-echo or half-Fourier acquisition single-shot turbospin-echo (HASTE) sequences [1–3]. Using a series of short scans, fetal MRI can be performed within the time constraints of a typical clinical imaging session without maternal or fetal sedation, with a total scan time that typically varies between 30 and 60 min. [1,4,5]. Fetal MRI is a helpful and safe secondary modality for confirmation and further characterization of fetal malformations suspected on US, as well as the detection or exclusion of additional pathology. It is most commonly performed for abnormalities of the central nervous system, diaphragmatic herniation, or neural tube defects [6, 7]. While the T2-weighted fast MRI sequence is considered to be the workhorse of fetal MRI, more and more sequences are being applied to fetal MRI that reflect development beyond pure morphology. Some of the techniques that have been adapted to in utero imaging include diffusion-weighted MRI [8], diffusion tensor MRI [9], susceptibility-weighted imaging [10], functional MRI based on the blood-oxygen level–dependent contrast [11], proton spectroscopy [12], and musculoskeletal imaging techniques [13].
The precise assessment of feto-maternal circulation, fetal viability, and the stage of functional maturity of fetal organs has central importance for decision making in obstetric practice. The depiction of the fine vasculature in fetuses is challenging, and therefore tissue viability is often assessed on the basis of indirect markers of tissue perfusion. Perfusion—the measure of blood circulation in the vascular bed per unit volume—can be characterized by the transit of various tracer compounds across living tissues, using either intravascular contrast agents or metabolic tracers. Perfusion computed tomography, nuclear medicine techniques, and contrast-enhanced MRI are nevertheless contraindicated for most pregnancies, and therefore, other noninvasive approaches are required to map tissue perfusion prenatally.
IVIM captures the randomized intrinsic displacements of fluids in tissues, which arise as a consequence of blood flow at the level of capillaries and short arterioles in living tissues [14]. Prenatal IVIM MRI is a particularly appealing method for assessing organ viability and the development of vasculature or other fluid spaces, and placental IVIM MRI in particular has recently gained popularity. The first studies in the early 2000s to utilize fetal IVIM MRI exclusively assessed placental development [15] and its pathologies, such as intrauterine growth retardation of placental origin [16–18]. The signal from the placenta remains mostly unaffected by the unpredictable movements of the fetus, and its volume is large enough to acquire reliable region of interest (ROI)-based IVIM estimates or even IVIM parametric maps. Placental IVIM MRI is discussed in a separate chapter of this book. In contrast to the placenta, several problems must be addressed before the imaging of the organs of the freely moving fetus with IVIM becomes clinically feasible.
To date, two studies have been published reporting nonplacental fetal IVIM MRI [19, 20], and our work group has conducted preliminary studies to estimate the repeatability and signal-to-noise ratio (SNR) of such data. Further works comprise that by Moore et al. [21], in which the biexponential model was fitted to multi-b-value fetal diffusion MRI data of the lung, but they concluded that the data were too noisy to resolve two diffusion components within the lung.
17.2 Fetal IVIM Acquisition
Due to the scarcity of studies utilizing low b values during prenatal diffusion-weighted imaging (DWI) or IVIM, there are no clear protocol recommendations. In this section we give an example on how to optimize IVIM MRI for fetal applications. We start by suggesting how to find the optimal b-value range and then propose ways of shortening the acquisition time to combat fetal motion.
It is crucial to consider the b value that separates the pure diffusion from the microvascular perfusion part of the signal. Unfortunately, we currently lack information about how this separation should be performed for the IVIM signal of developing organs. The brains of neonates and young infants have higher water content than adults, which is associated with longer T2- and higher apparent diffusion coefficient (ADC) values. It is safe to assume that the water content of fetal organs is higher than that of the infant or adult organs and so lower maximal b values might be sufficient. It is typical to use 600–700 s/mm² during DWI of the fetal brain [22], which may also mark the meaningful maximum b value for fetal IVIM. Data dropout is considerable, and according to our experience, up to 25%–50% of the image frames may have to be removed due to motion-induced artifacts. In our current practice, corrupted images are marked manually and are removed together with the corresponding entry from the b matrix. We suggest avoiding signal averaging during the acquisition (i.e., by using a higher number of averages/excitations) and propose acquiring multiple images with partial k-space sampling and averaging during postprocessing after corrupted images have been removed.
These considerations lead us to the conclusion that it is worth using multiple repetitions for each set of b values such that sufficient data remain after removing up to 50% of the frames. In recent works by Jakab et al. [19, 20], the following acquisition parameters were used for fetal IVIM MRI, which were implemented for 1.5 T and 3 T scanners.
- MR coil: A 32-channel phased array cardiac coil or 8-channel body flex coil according to maternal body habitus. The coil is positioned according to the expected location of the relevant fetal structures.
- Sequence: Dual spin-echo echo-planar sequence, TE/TR: 2200/75 ms.
- Diffusion-weighting scheme: Tetrahedral gradient encoding scheme, one b0 image, b-value range: 10, 20, 30, 40, 60, 80, 100, 150, 200, 300, 400, 500, 600, 700, 800, and 900 s/mm2.
- Positioning and image dimensions: Image matrix, 80 * 100; resampled to an in-plane voxel size of 0.5 * 0.5 mm; number of slices, 8–14; slice thickness, 3 or 4 mm; and slice gap, 0.5 mm. Slice orientation and positioning are adjusted to cover the relevant fetal organ with the fewest possible slices.
- Imaging time: 1 min. 40 s to 3 min. 20 s.
- Diffusion-weighting scheme: Tetrahedral gradient encoding scheme, one b0 image, b-value range: 10, 20, 30, 40, 60, 80, 100, 150, 200, 300, 400, 500, 600, 700, 800, and 900 s/mm2.
17.3 Motion Correction
Fetal IVIM is particularly affected by two different types of dynamic signal changes within the images. First, spatial artifacts are induced due to fetal motion, maternal breathing, and physiological pulsations. The fetus is not sedated during the MRI. Therefore, fetal motion cannot be controlled. Second, image contrast is dynamically changing with the increasing b values. When correcting for motion, both processes have to be taken into consideration. The problem of the changing image contrast as a function of the b value might be resolved by using normalized gradient fields during nonlinear motion correction or with the progressive principal component registration method [23, 24]. One way to counteract motion is to shorten the effective acquisition time by using the fewest possible slices.
17.3.1 Motion Correction and Image Reconstruction Methods
The retrospective correction of motion by image processing appears to be more challenging for fetal IVIM than for other fetal imaging modalities. IVIM most commonly uses echo-planar imaging, which acquires several 2D slices, each in a single shot, over a 3D imaging volume. Fetal movement can occur in the direction that is perpendicular to the imaging plane before the complete acquisition of all slices. This means that there is fetal motion present between the slice acquisitions, leading to a distortion in the anatomy of the fetus.
The latter problem is addressed by reconstruction approaches that are commonly used to generate higher resolution images from a series of lower-resolution 2D acquisitions (= “super-resolution” [25]). A further complication of fetal movement is the spin history artifact, which can partially be tackled by outlier rejection methods during the image reconstruction [26]. Slice-to-volume (SVR) approaches inject individual 2D slices into the image registration process to tackle between-slice movements and to achieve a higher resolution [27]. The most common application of fetal imaging using SVR is the brain, as this method can only be used for correcting motion of rigid objects.
Surprisingly, in fetal DWI, diffusion tensor imaging (DTI), and IVIM, the brain appears to undergo nonlinear spatial distortions as well as linear distortions due to the complex chemical environment that leads to unpredictable susceptibility-related shifts in the image. Other organs may be shifted relative to each other, and they are not encaged by rigid skeletal structures. It was suggested that patch- to-volume reconstruction (PVR) methods can potentially tackle such nonlinear distortions in nonbrain fetal imaging [28]. PVR is a more recent super-resolution method that is able to reconstruct a large field of view of nonrigidly deforming structures using a parallelized patchwork optimization (patch-to-volume registration is used instead of slice-to-volume registration) and automatic outlier rejection. In both approaches, robust statistics are used to identify improperly registered data or data that are heavily corrupted by artifacts [8]. The use of such advanced postprocessing methods has been suggested for fetal diffusion MRI [29, 30]. However, to the best of our knowledge, no study exists that has applied SVR or PVR for IVIM data sets. With the currently clinically available scan times it is an unrealistic expectation to acquire multiplane, thin-slice IVIM of the whole fetal body for SR reconstruction. A realistic compromise is to have thicker slices and choose an acquisition plane that depicts the anatomy of the pathology with fewest possible slices, such as coronal imaging for fetal body pathologies. The nonlinear distortion could be tackled by frame-by-frame image registration using elastic or fluid registration approaches, as has been suggested for compensating the breathing motion in different imaging modalities [31, 32]. However, it might become necessary to regularize the deformation fields to allow for physically realistic organ motion in the human body [33]. We utilized a free-form deformation algorithm for nonrigid registration, which was implemented as the reg_f3d command in the NiftyReg software [34] and which was found to be able to compensate for smaller shifts of thoracic and abdominal fetal organs.
17.3.2 Tracking Organ Motion Using Image Registration Methods and Artificial Intelligence
IVIM benefits from a higher SNR, which, depending on the clinical question, is feasibly achieved by fitting the model to intensity values that have been averaged over larger volumes of interest (VOIs). An example of fetal IVIM MRI acquisition is shown in Fig 17.1, and the mean signal over a VOI is demonstrate in Fig 17.1(e). The precise segmentation of whole fetal organs is important for this VOI-based approach. As discussed in this chapter, images are affected by nonlinear shifts, which necessitate the delineation of the VOI for each image frame, as motion compensation with postprocessing might not be perfect. The outlines of organs on medical images can be segmented using machine learning approaches, and this can be used to track the trajectory of organs in the presence of motion. For the fetal brain, the deep convolutional neural network method was suggested to overcome the object detection and tracking problem [35]. Image registration using the optical flow approach may also be useful for this purpose: here, motion is estimated by assuming that image pixels conserve their intensity along their trajectory, to which a spatial regularity constraint of the estimated motion is added [36].
Figure 17.1 A fetal IVIM MRI. (a) Acoronal whole-body acquisition, T2- weighted image, (b) four frames of the IVIM imaging sequence illustrated with increasing b values, (c) a T2-weighted image of the lung of a fetus at the 30th week of gestation, diagnosed with congenital pulmonary airway malformation (CPAM), (d) a microvascular perfusion fraction (f) map (*: CPAM showing decreased f values), and (e) IVIM signal characteristic over the contralateral, unaffected lung, corresponding to the averaged values inside the red outlines.
17.4 Quality of Fetal IVIM MRI
In a reproducibility study [20], we found that 6.1 ± 7.4 out of 64 image frames with excessive motion had to be removed before the analysis. The reproducibility of the f, d, and D* over the repeated scans were given as the test-retest variability (VAR%). We found that the reproducibility of the diffusion coefficient was the highest for all the investigated fetal structures and the placenta (VAR%; frontal cortex, 4.8%; placenta, 12.2%), as estimated by the monoexponential decay of signal intensities corresponding to image frames with a b factor >250 s/mm2. In contrast, f and D* values were twice as variable across repeated scans as the diffusion coefficient. Only three organs, namely the placenta, the fetal liver, and the fetal lungs, were found to show moderately reproducible perfusion fractions and pseudodiffusion coefficients, while the f and D* of the investigated brain area and kidneys showed poor reproducibility, which was defined as a test-retest variability of over 25%.
IVIM is particularly sensitive to noise, and a high SNR is required for the reliable estimation of the microvascular perfusion fraction. Noise is particularly prominent at higher b values for which the SNR becomes low. We estimated the SNR of IVIM MRI acquisitions for the placenta of five fetuses between the 22nd and 36th week of gestation. For these subjects, the same IVIM sequence was repeated twice in consecutive scans. The SNR was measured for four different b values (b = 0, 150, 700, and 900 s/mm2) using the National Electrical Manufacturers Association (NEMA) subtraction method applied to within-subject repeated IVIM scans [37]. We found the following SNR values: b = 0 s/mm2 images, 15.0 ± 7.0 (10.2–27.2); b = 150 s/mm2 images, 10.5 ± 3.2 (8.0–16.0); b = 700 s/mm2 images, 6.4 ± 1.3 (4.6–7.8), and for the b = 900 s/mm2 images, 4.5 ± 2.0 (2.4–7.7).
17.5 IVIM MRI of Fetal Organ Maturation
Fetal MRI is typically indicated and performed during the second and third trimesters of gestation. During this period, organs undergo a rapid maturation process. In addition to their apparent quantitative change—volume growth—crucial events take place that enable physiological function after birth and greatly determine the viability of the fetus in the case of premature birth.
Fetal lungs undergo significant microstructural development during mid- and late gestation, marking the maturation of the airways and vasculature. The surfactant producing and storing ability of type II alveolar cells of the lungs has long been known to determine not only survival but also any urgent need for medical intervention. Fetal airways contain no air, and imaging by MRI, especially diffusion-weighted MRI, is technically feasible [38]. A currently accepted marker of maturity and predictor of outcome in certain diseases, such as in congenital diaphragmatic hernia, is lung volume, which is relatively easy to measure in T2-weighted MRI [39]. In contrast, the signal intensity in T2-weighted imaging or ADC values showed only weak or no correlation to surfactant levels [40, 41].
The fetal lungs demonstrate rapid changes of the diffusion coef- ficient with development [42]. Fetal IVIM MRI can potentially shed light on this maturation process. We found increasing microvascular perfusion fraction of the fetal lungs during gestation [19], and fetus- es older than the 32nd gestational week demonstrated a 72% higher f than those between the 17th and 31st weeks. To better understand how ADC and f values change over gestation, multiple coexisting compartments in the lungs have to be considered. The IVIM signal is likely to be influenced by vascular development. The ingrowth and proliferation of capillaries in the lung parenchyma begins in the canalicular phase (until the 26th week), and the development of the vasculature and increase in arterial blood flow parallels the arborization of the airways. After the 26th week, the saccular phase begins, arborization continues, and surfactant production takes place. The airways are filled with free-diffusing and actively secreted fluid, and the diameter and apparent segment lengths of these airways also change dynamically. Due to the relatively large volume of the fetal lungs, especially at the critical period of fetal lung development at the beginning of the third trimester, fetal IVIM MRI may play an im- portant role in the diagnostic workup.
The fetal liver is easily recognized in an MRI, and its large relative volume makes it a good candidate to be examined with IVIM MRI. The liver also undergoes rapid gestational change, and the fetal liver and its complex network of vessels play an important role in fetal circulation, which gradually adapts to the growing body mass. The proportion of arterial blood that is shunted away from the liver via the ductus venosus gradually decreases as the fetus grows. We revealed a significantly decreasing microvascular perfusion fraction in the fetal liver with an increasing gestational age [19], which may reflect a vascular process, which is a consequence of the reduction of weight-normalized blood supply and venous drainage through the umbilical vessels. This assumption is supported by the large blood content of the liver: blood volume accounts for one-third of the water content in liver, and the greatest contributor to blood flow is the small vessel content.
17.6 Clinical Cases
Fetal IVIM MRI has not yet reached clinical maturity due to different factors. First, we lack normative data on how the IVIM metrics change during gestation and how they reflect the maturation of tissue microstructure. Data dropout and the need for complicated post processing may also delay clinical translation and render fetal IVIM to be a specialist method that is performed for select cases only. Here we demonstrate possible applications for fetal IVIM in selected pathologies.
17.6.1 Vein of Galen Aneurysmal Malformations
Vein of Galen aneurismal malformations are the most frequent arteriovenous malformations in fetuses. Fetal MRI of the brain may provide novel information in addition to the US: first, to depict the 3D anatomy of the dilated vessels that are supported by arteriovenous fistulas, and second, to demonstrate early complications, such as hydrocephalus, cerebral ischemia, or hemorrhage. The turbulent flow within the aneurysm may mimic incoherent intravoxel dephasing, which leads to a distribution of velocities and an isotropic IVIM signal attenuation that is similar to, or even larger than, the perfusion-driven component of the IVIM signal (Fig 17.2). This way IVIM angiograms could be generated without the use of contrast agents and voxels can be identified with excessive flow compared to their environment, which is expected in the presence of feeding vessels.
Figure 17.2 A fetus at the 31st week of gestation, diagnosed with aneurysmal malformation of the vein of Galen. (a) An axial T2-weighted MRI showing the aneurysmatically dilated veins, (b) an axial DWI at the level of the aneurysm, (c) an ADC map, (d) a microvascular perfusion fraction (f) map, calculated from IVIM MRI, overlaid after masking with the b0 image, and (e) the IVIM signal from a single voxel in the center of the vein Galen aneurysm; the calculated f is 0.6.
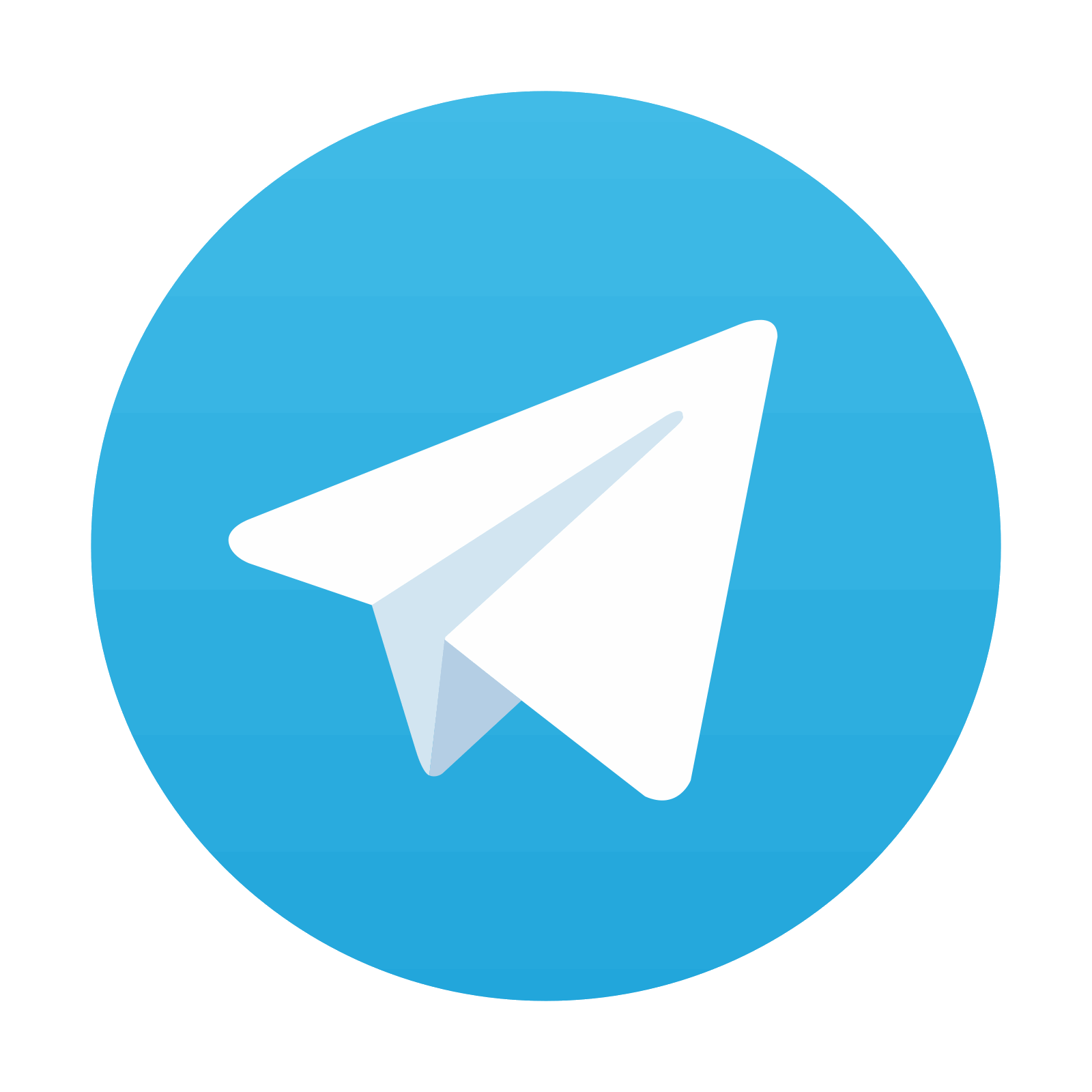
Stay updated, free articles. Join our Telegram channel
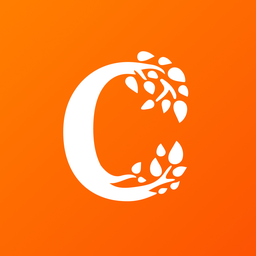
Full access? Get Clinical Tree
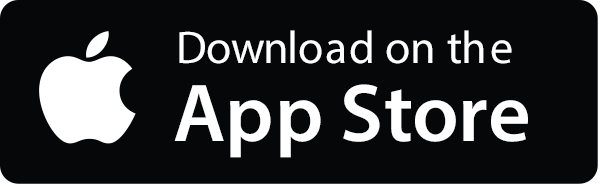
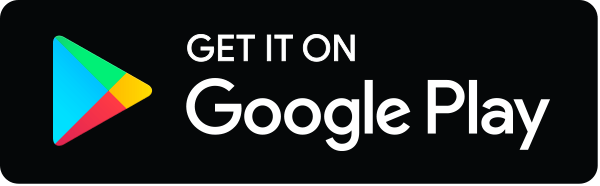