, Ahmad Ameri1 and Mona Malekzadeh2
(1)
Department of Clinical Oncology, Imam Hossein Educational Hospital, Shahid Beheshti University of Medical Sciences (SBMU), Shahid Madani Street, Tehran, Iran
(2)
Department of Radiotherapy and Oncology, Shohadaye Tajrish Educational Hospital Shahid Beheshti University of Medical Sciences (SBMU), Tehran, Iran
Profound hematologic toxicity, prominently leukopenia, frequently develops following radiation therapy that includes a large volume of bone marrow in the radiation field [1–8]. The percentage of patients with radiation-induced leukopenia has been reported in up to 50% and 90% of patients treated with pelvic field irradiation alone and with concurrent chemotherapy, respectively [1, 6]. These values are lower for thrombocytopenia (1% and 30% of patients treated with pelvic field irradiation alone and with concurrent chemotherapy, respectively) and anemia (30% and 50% of patients treated with pelvic field irradiation alone and with concurrent chemotherapy, respectively) [1, 10].
19.1 Mechanism
The bone marrow is a cellular stroma consisting of the vascular system with nutritive vessels and a very complex sinusoidal system [11]. At birth, virtually all marrow is hematopoietic. Conversion of red marrow to yellow marrow with advancing age begins in the distal bones, and the distribution of active red marrow in the adult is in the skull (13.1%), clavicle (1.5%), scapula (4.8%), sternum (2.3%), ribs (7.9%), vertebrae (28.4%), pelvis (36.2%), and proximal extremities (5.7%). There is a gradual reduction in the percentage of cellularity of red marrow with age and its replacement by fatty yellow marrow [12].
The cellular stroma of bone marrow houses active blood-cell-forming stem cells [13]. The peripheral blood also contains hematopoietic stem and progenitor cells. Due to the dynamic equilibrium between the hemopoietic cell populations in the bone marrow and peripheral blood, the peripheral blood cell populations could be sensitive indicators of radiation-induced damage to hematopoietic tissues [14].
The hematopoietic stem and progenitor cells are highly sensitive to radiation [15]; however, nondividing mature cells (e.g., granulocytes, red cells, or platelets) tolerate higher radiation doses. After exposures at doses as low as 4 Gy, the cellularity of the irradiated bone marrow progressively declines [16–18]. It has been found that an exposure dose of 30–47 Gy delivered in a period of 3–4 weeks to the sternal area results in severe depression of the sternal marrow [19].
Breakdown of the sinusoidal system results in erythrocytes spreading throughout the parenchyma, and a hemorrhagic bone marrow develops with radiation [16].
The most sensitive indication of acute radiation effect on peripheral blood counts is a reduction in number of circulating lymphocytes, together with the appearance of abnormal lymphocyte figures [17]. If the limited volume of marrow receives radiation (less than 3% of the total active adult marrow), the peripheral blood counts may be within normal limits despite marrow hypoplasia [17].
It has been demonstrated that irradiated bone marrow sites can be recovered early by the stem cell migration of the protected bone marrow sites or peripheral blood [13, 20, 21]. Proliferation and release of stem cells increase in non-irradiated bone marrow after radiation therapy. The depletion of stem cells in the protected bone marrow at the beginning of radiotherapy seems to be due to such migration [16, 22]. Another reason for this diminution could be the result of an increased rate of differentiation.
After stem cell transplantation, the times to “rebuild” for the granulocytic, erythropoietic, and megakaryocytic cell lines are 10–12, 5–7, and 10 days, respectively [11].
Although blood count recovery occurs a few months after completion of therapy in 90% of patients, bone marrow regeneration and recovery require a much longer time. The potential of regeneration appears to depend on the dose received. It has been shown that a dosage of 30 Gy or more of bone marrow radiation needs an extended time to recover and may permanently depress the marrow [19, 23]. This could be due to permanent damage to the precursor cells of the marrow elements or to a physiological alteration of the blood vessels and supporting syncytium. These factors could be responsible for the prevention of growth of the regenerating cells and/or the repopulation of cells from marrow in other parts of the body [17]. Permanent loss of hematopoietic activity in the irradiated areas is compensated by increased activity in the non-irradiated areas [24, 25].
19.2 Timing
In patients undergoing conventional fractionated radiation therapy, occurrence, degree, and timing of blood count suppression are dependent on the proportion of active marrow volume in the radiation fields. The field size is the most important determining factor for the adverse hematologic effects on severity and timing. For example, it is estimated that the proportion of active marrow included in the periaortic, mantle, and pelvic fields is about 10%, 25%, and 40%, respectively, and nadir levels of the cell counts occur at different times for these fields [26].
The number of peripheral blood cells decreases according to their radiation sensitivity and life expectancy. The total white cell count declines during radiation therapy. Lymphocytes are the most sensitive, and monocytes are the most refractory leukocytes to change. The lymphocyte count drops first and by the greatest amount, the neutrophils less, and the monocytes only exhibit a transient, early, modest dip. After the nadir, counts for white cells levels maintain a plateau [27].
The platelet count declines gradually with radiation therapy to a nadir and thereafter is maintained its level [27].
The hemoglobin very gradually decreases during radiation therapy and reaches its lowest value at the end of radiation therapy, which could be related to the long red blood cell life expectancy compared to leukocytes and platelets [27].
Complete peripheral blood count recovery is observed at 1–3 months following therapy, although it may still be below the pre-therapy levels, and lymphocyte count recovers fastest [27]. Peripheral blood counts are an unreliable indicator for assessing the true status of bone marrow reserve, and bone marrow suppression may sustain for months to years despite normalized peripheral blood counts [28, 29]. Patients may have normal peripheral blood counts with a marked suppression of bone marrow secondary to increasing proliferation of unexposed bone marrow [28].
19.3 Risk Factors
Radiation-related factors such as larger radiation field and higher radiation dose are the most important factors affecting bone marrow injury and degree of depression in the peripheral blood counts [19, 30].
Chemotherapy is another factor affecting hematologic toxicity of radiation therapy. Patients with previous and concomitant chemotherapy have increased risk for radiation-induced neutropenia [31].
19.4 Symptoms
Depression of each hematopoietic cell lineage translates into potential problems for the patient. The Radiation Therapy Oncology Group has defined a scoring system for acute hematologic toxicity of radiotherapy (Table 19.1) [9].
Table 19.1
RTOG acute radiation hematologic effects scoring
Grade 1 | Grade 2 | Grade 3 | Grade 4 | |
---|---|---|---|---|
White blood cells/ml | 3000 to <4000 | 2000 to <3000 | 1000 to <2000 | <1000 |
Platelet/ml | 75,000 to <100,000 | 50,000 to <75,000 | 25,000 to <50,000 | <25,000 or spontaneous bleeding |
Neutrophils/ml | 1500 to <1900 | 1000 to <1500 | 500 to <1000 | <500 or sepsis |
Hgb (g/dl)/Hct(%) | 9.5 to <11/28 to <32 | 7.5 to <9.5/ <28 | 5 to <7.5 (pack cell transfusion required) | – |
Patients with isolated neutropenia may not have any specific symptoms. Neutropenia may place the patient at substantial risk for infection, and a fever may be the first sign.
Thrombocytopenia is generally not associated with any symptoms and is self-limited, but manifestations of bruising, petechiae, and mucosal bleeding infrequently occur. Intracranial hemorrhage is the most dangerous manifestation of thrombocytopenia, though it is particularly rare, and most episodes occur at platelet counts less than 5000/mL.
Symptoms related to significant anemia are easy fatigue, low compliance for activity, rapid heart rate, and shortness of breath.
19.5 Prevention
There is correlation between radiation technique parameters regarding bone marrow and hematologic toxicity. These parameters could be modifiable with intensity-modulated radiation therapy (IMRT) and more advanced techniques. New techniques can reduce radiation dose to bone marrow and decrease acute hematologic toxicity [32–35].
One problem with standard IMRT planning is the large volume of bone marrow that needs to be avoided. By considering only red (active) marrow in marrow contouring, the planning process could be improved [36]. The distribution of marrow containing red (active) and yellow (inactive) marrow is different among individuals [37]. Active red marrow can be visualized poorly in computed tomography (CT) scanning. Functional bone marrow imaging like 2-deoxy-2-[F-18]fluoro-D-glucose (FDG)-positron emission tomography (FDG-PET) [38] or single-photon emission computed tomography (SPECT) [18] can provide information about functional normal bone marrow and its physiologic distribution. Incorporation of these modalities into the IMRT planning process avoids the unnecessary contouring of inactive yellow marrow for calculation of dose constraints. Structural imaging techniques such as magnetic resonance imaging (MRI) in place of functional imaging may be useful in this regard [18, 37, 38].
The potential for cytokines in prevention of the hematopoietic system radiation injury has been studied in lethally irradiated animals, and observations suggest that some cytokines such as G-CSF [39, 40], interleukin-1 (IL-1) [41, 42], tumor necrosis factor (TNF)-alpha [43], and Fms-related tyrosine kinase 3 ligand (FLT3LG) [44–46] may mediate radioprotective effects to the hematopoietic progenitor cell and stem cell compartments. It has also been shown that meloxicam, a selective inhibitor of cyclooxygenase 2, can modulate hematopoiesis and elevate numbers of granulocytic precursor cells in bone marrow and granulocyte counts in peripheral blood by increasing endogenous G-CSF production [47]. Currently, there is no recommendation for the prophylactic use of these factors.
19.6 Treatment
19.6.1 Neutropenia
The proliferation and differentiation of hematopoietic cells are regulated by a family of cytokines including colony-stimulating factors (CSFs) and interleukins, which are specific to each cell lineage [48]. Granulocyte colony-stimulating factor (G-CSF) controls the production, differentiation, and function of neutrophilic granulocytes. Single classes of high-affinity receptor for G-CSF are present on the precursor and mature cells of neutrophilic granulocytes [48].
The mature human G-CSF is a 19.6 kDa glycoprotein containing 174 amino acids [49], and its molecular cloning has been well described [50].
It is well known that E. coli is a useful host for the production of recombinant human G-CSF [51], but there are also various products that are derived from different tissues (e.g., yeast, transgenic plants, and mammalian cells) [52].
There are lots of controversies about using of colony-stimulating factors during radiation therapy regarding acute and late side effects.
G-CSF treatment is well tolerated during continuous fractionated radiation therapy and can be used clinically to alleviate severe and threatening neutropenia caused by radiation therapy or by combined radio-chemotherapy [53, 54].
The number of G-CSF receptors on bone marrow cells increases with radiation [55], and administration of recombinant human G-CSF can accelerate the recovery of radiation-induced neutropenia [56–58] and reduce its severity and duration [59–62].
After administration of recombinant human G-CSF, the level of circulating neutrophils increases by accelerating production, inhibition of neutrophil apoptosis, reduction of transit time from stem cell to mature neutrophil, and accelerating neutrophil entry into the blood, and neutrophil function is enhanced. An increase in neutrophil progenitors in the marrow and hematopoietic stem cell mobilization can be seen in G-CSF administration [63–65].
Clinical application of recombinant human G-CSF is hampered in some situations by various side effects. G-CSFs should not be included in patients receiving concomitant chemotherapy and radiation therapy, particularly involving the mediastinum based on the results of GM-CSF or G-CSF morbidity in mediastinal chemoradiotherapy. More severe thrombocytopenia was seen in patients with CSF administration [66–68]. Further studies will be required to determine if chemoradiotherapy for other sites of disease will provoke similar problems.
Possible reason for thrombocytopenia observed in this site of irradiation is that the CSF mobilizes progenitor cells into the peripheral blood and new precursor cells are destroyed during migration into the irradiation volume because large numbers of these cells may be irradiated as they pass through the heart [67].
Outside of clinical trials, in patients receiving concomitant chemotherapy and radiation therapy, treatment is suspended for severe neutropenia and is resumed when the toxicity decreases to lower grade.
There is a concern about the reduction in the capacity of bone marrow recovery with simultaneous treatment of G-CSF during radiation therapy in the postradiation phase. Mechanisms like enhanced production of inhibitory cytokines, a decrease in endogenous production of the growth factors due to the exogenously induced pharmacological levels, downregulation of growth factor receptors on target cells [69], and decreased sensitivity of bone marrow to radiation during G-CSF application are proposed for this phenomenon [70]. Further investigations are needed to determine the late bone marrow effect of G-CSF administration during radiation therapy and the timing, duration, and dosage of G-CSF application for successful treatment and a favorable outcome.
The American Society of Clinical Oncology (ASCO) guidelines recommend that “CSFs should be avoided in patients receiving concomitant chemotherapy and radiation therapy, particularly involving the mediastinum. In the absence of chemotherapy, therapeutic use of CSFs may be considered in patients receiving radiation therapy alone if prolonged delays secondary to neutropenia are expected.”
European Society of Clinical Oncology (ESMO) guidelines also recommend that “primary prophylaxis with G-CSF is not indicated during chemoradiotherapy to the chest due to increased rate of bone marrow suppression associated with an increased risk of complications and death” [71].
Three clinically available forms of G-CSF are [72]:
*A glycosylated form (lenograstim), which is produced by using the expression in mammalian cells, and a *non-glycosylated form (filgrastim), which is produced by using expression in E. coli [73]
*A pegylated form of non-glycosylated Hu G-CSF (pegfilgrastim)
Pegfilgrastim is developed to produce a long-acting filgrastim requiring less frequent dosing than its parent drug [74]. A single dose of PEG-rhG-CSF has a similar clinical safety to rhG-CSF and significant advantageous effects [75].
The recommended dose for G-CSF is 5 g/kg/day and for Pegfilgrastim is as a single dose of either 100 mcg/kg (individualized) or of a total dose of 6 mg (general approach) [71, 76]. Both are injected subcutaneously. A single subcutaneous injection of rhG-CSF results in an increase in circulating granulocytes within 2 h; the granulocyte concentration peaks by 12 h and remains elevated for the next 36 h before decreasing slowly back to baseline [77]. The median time to reach the peak concentration is 2.5–5 days for pegfilgrastim, and neutrophilic level remains above baseline for around 9–10 days [74].
Patients should be monitored with serial CBCs. Administration should be continued until the absolute neutrophil count reaches to upper level or normal [78].
The G-CSF receptors are expressed not only on myeloid cells but also on other hematopoietic cells including monocytes, platelets, and possibly certain lymphocyte subsets [79]. No significant changes in lymphocyte or monocyte counts have been observed during the course of rhG-CSF treatment.
Data on the effects of G-CSF on platelet function are limited. It seems that G-CSF enhances platelet aggregation and activation in humans [80].
Radiation-induced thrombocytopenia may be worsened by G-CSF administration, possibly due to a decrease in the number of megakaryocytes in the bone marrow and an increase in the trapping of megakaryocytes in the spleen [72, 81]. G-CSF-induced thrombocytopenia seems to be a transient event and may improve spontaneously despite continual G-CSF treatment [82].
Common side effects observed during G-CSF treatment consist of mild musculoskeletal pain, papular skin rash, and some laboratory abnormalities like elevated alkaline phosphates [54, 61, 82].
The exact effects of G-CSF administration on cancer cells remain controversial. G-CSF administration increases the production of neutrophils, and cytotoxic mediators produced by neutrophils could kill the cancer cells [83, 84]. On the other hand, G-CSF has been shown to promote tumor angiogenesis by upregulating VEGF [85], which is released by neutrophils and reduces radiation-induced vascular damage [86].
It has been shown that prophylactic G-CSF administration for treatment of advanced unresectable head and neck cancers resulted in an unexpected reduced local control [87, 88]. The effects of G-CSF on tumor growth, especially during radiation therapy, are controversial and require further investigation [84].
Furthermore G-CSF can stimulate the clonal growth of some non-hematopoietic tumor cells like bladder [89] or colon [90] cancers, and the remote effect of G-CSF on the growth of tumor cells lying outside radiation treatment portals is proposed by some [91].
It has been suggested that the simultaneous or sequential administration of two or more complementary cytokines has resulted in better patterns of hematologic recovery. The capacity of multiple cytokine combinations (like GM-CSF and IL-3 [92–94], TPO with IL-4 or IL-11 [95], IL-3 receptor agonist and rhG-CSF [96], and rhG-CSF and pegylated megakaryocyte growth and development factor [97]) to accelerate hematologic recovery and ensure multi-lineage protection following severe radiation-induced myelosuppression has been assessed in animal studies with promising results [92–98]. Further investigations in this field will clearly be necessary before drawing any conclusions.
19.6.2 Thrombocytopenia
There is no threshold for platelet counts that for counts below which prophylactic transfusion should be prescribed to all patients with radiation-induced thrombocytopenia. Several studies support a threshold of 10,000 platelets/mL or less for initiating transfusions to patients with solid tumors and a higher threshold; perhaps 20,000/mL has been proposed for patients with tumor with the presence of necrotic sites (e.g., gynecologic, colorectal, melanoma, or bladder tumors) [23, 99].
Thrombopoietin, a lineage-specific growth factor specific for platelets, regulates platelet production via the stimulation of its receptor on megakaryocytes and platelets leading to proliferation and differentiation. Two variant forms of human thrombopoietin have been developed including first-generation agents, recombinant human thrombopoietin (rHuTPO) and pegylated recombinant human megakaryocyte growth and development factor (PEG rHuMGDF), and second-generation agents—the TPO peptide mimetics, TPO non-peptide mimetics, and TPO agonist antibodies [100–102].
Clinical trials with recombinant TPO [100] and recently romiplostim (a TPO peptide mimetic) [103] have shown that these agents can improve platelet counts in patients receiving chemotherapy. Further studies need to be performed for use of these agents in radiation-induced thrombocytopenia.
Platelet factor 4 (PF4) is released from radiation-damaged megakaryocytes and inversely affects platelet count recovery after radiation. Anti-PF4 strategy can improve outcomes of radiation-induced thrombocytopenia and needs to be explored [104].
Interleukin 11 (IL-11) is a stromal cell-derived cytokine that enhances the growth of early progenitors and promotes megakaryocytopoiesis and erythropoiesis [105]. Given recombinant human interleukin-11 (rHuIL-11) activity in chemotherapy-induced thrombocytopenia, it needs to be studied as one possible therapeutic option in radiation-induced thrombocytopenia [106–108].
19.6.3 Anemia
Radiation is more effective on tumor cells with higher oxygenation. Anemia is associated with poor tumor oxygenation, relative tumor radioresistance, and decreases the effectiveness of radiation therapy, with subsequent reductions in treatment outcomes including locoregional control and overall survival of cancer patients [10, 109]. Correcting the radiation-induced anemia and maintaining normal hemoglobin levels in patients undergoing radiation therapy have a significant importance due to decrease in tumor hypoxia, improve treatment outcomes, and have a determinant impact on quality of life [109–112]. Both blood transfusion and use of erythropoiesis-stimulating agents (e.g., darbepoetin, erythropoietin) can increase the hemoglobin levels during radiation therapy, but the effects of medications used for correction of radiation-induced anemia on locoregional control and overall survival are questionable [113–117].
Many radiation oncologists have not given ample attention to treating mild to moderate anemia during radiation therapy unless symptoms of severe anemia are present or the hemoglobin falls below a threshold of 9–10 g/dL. However, managing mild to moderate anemia is important for radiation therapy outcome improvement and quality of life preservation [109, 118].
Radiation-induced anemia is usually mild and readily correctable. It has been reported that for most patients with uterine and cervical cancer, the severity of anemia during radiation therapy was modest (59% of anemic patients with hemoglobin levels between 10 g/dL and 11.9 g/dL and 11% with hemoglobin levels between 9 g/dL and 9.9 g/dL). Only a few patients had levels below 8.9 g/dL. Data on colorectal, prostate, lung, and breast cancers yielded similar results [109].
Blood transfusion and recombinant human erythropoietin (rHuEPO) administration can significantly improve radiation-induced anemia [111, 113, 119–122]. There are no randomized studies comparing blood transfusion and rHuEPO efficacy and safety in patients undergoing radiation therapy.
A number of studies have shown an effect of perioperative transfusion on cancer recurrence rates [123–128]. The mechanism of how blood transfusion affects tumor viability is related to some types of immunosuppression [129]. Therefore, some have proposed that rHuEPO administration in patients undergoing radiation therapy may have some benefits over transfusion due to its potential to correct anemia without the risks associated with transfusion [130].
Several studies using transfusion to correct radiation-induced anemia have shown improvement in local control, but there is no study comparing transfusion with rHuEPO, and no definitive conclusion can be drawn about transfusion or rHuEPO safety during radiation therapy.
It has been demonstrated that erythropoietin receptors are expressed in several human cancer cells and may modulate the cellular effects of recombinant human erythropoietin on cancer cells, leading to increased cell survival and growth [131, 132]. Belenkov et al. reported that the addition of exogenous recombinant human erythropoietin induces cancer cells to become more resistant to ionizing radiation and to cisplatin [133]. These data indicate that the administration of rHuEPO to cancer patients undergoing radiation therapy should proceed with caution, especially when the cancer type has been shown to be positive for erythropoietin receptor.
There is no guideline for erythropoietin use during radiation therapy. Based on these findings, we recommend that erythropoietin should not be administered during radiation therapy outside of the experimental setting.
References
1.
Peters WA, Liu P, Barrett RJ, Stock RJ, Monk BJ, Berek JS et al (2000) Concurrent chemotherapy and pelvic radiation therapy compared with pelvic radiation therapy alone as adjuvant therapy after radical surgery in high-risk early-stage cancer of the cervix. J Clin Oncol 18(8):1606–1613PubMed
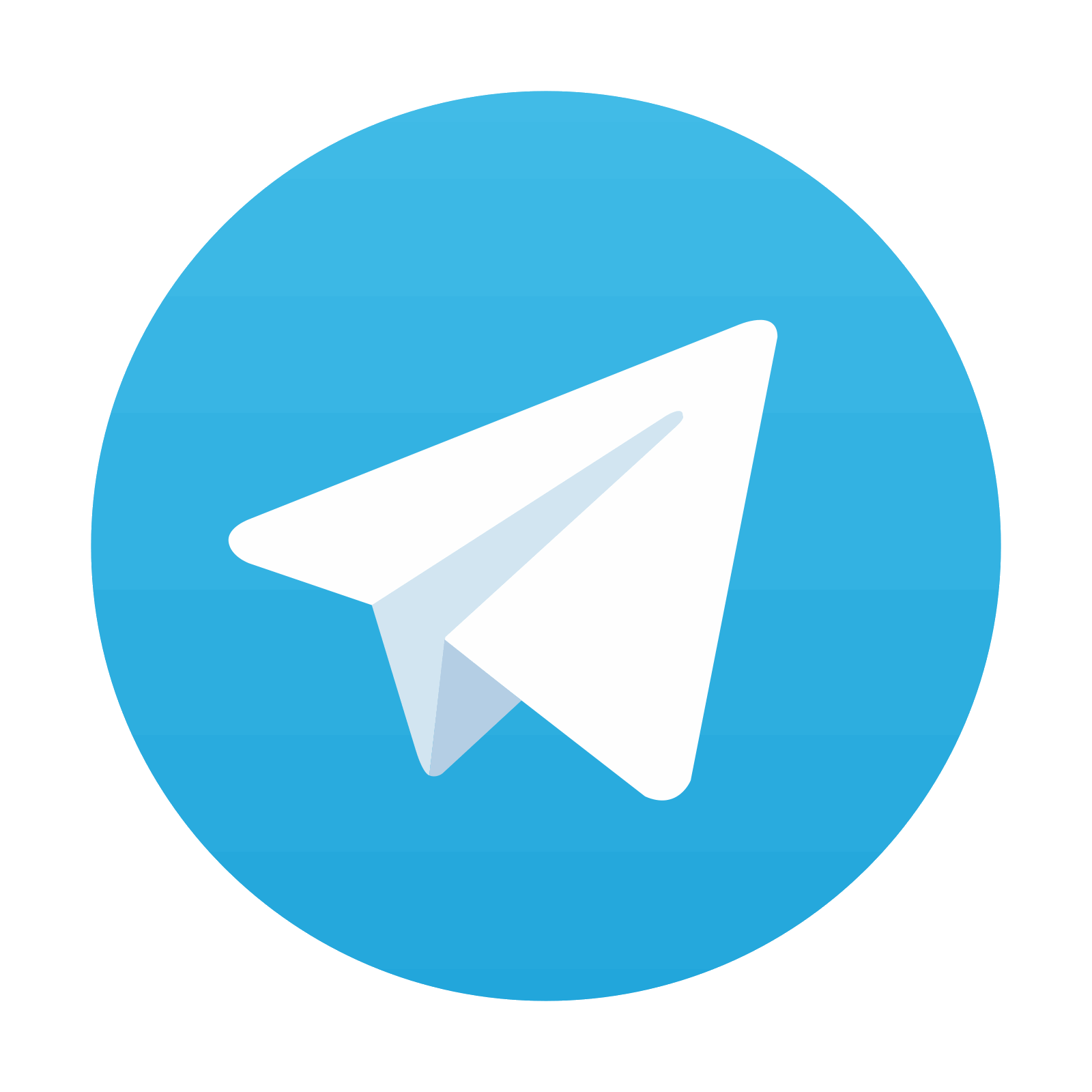
Stay updated, free articles. Join our Telegram channel
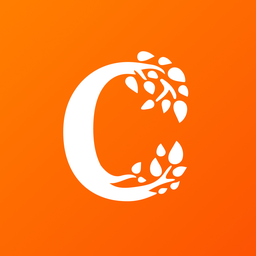
Full access? Get Clinical Tree
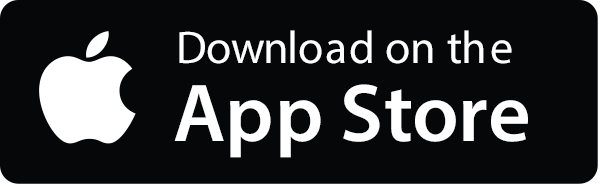
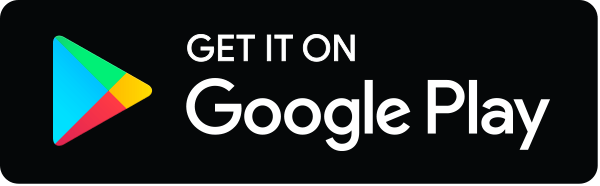
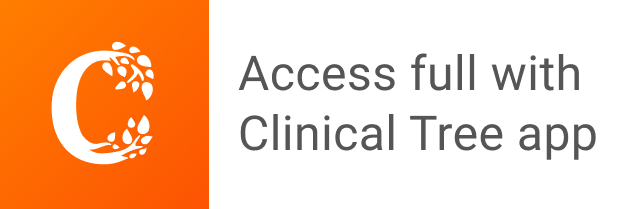