Image-guided tumor ablation is a minimally invasive strategy to treat focal tumors by inducing irreversible cellular injury through application of thermal and, more recently, nonthermal energy or chemical injection. This approach has become a widely accepted technique, incorporated into the treatment of a range of clinical circumstances, including treatment of focal tumors in the liver, lung, kidney, bone, and adrenal glands.1–4 Benefits of minimally invasive therapies compared to surgical resection include lesser mortality and morbidity, lower cost, and the ability to perform procedures on outpatients who are not good candidates for surgery.5 However, additional work is required to improve outcomes and overcome limitations in ablative efficacy, including persistent growth of residual tumor at the ablation margin, the inability to effectively treat larger tumors, and variability in complete treatment based upon tumor location.1 The overall goal of minimally invasive focal tumor ablation encompasses several specific aims. First and foremost, a successful ablation completely treats all malignant cells within the target. This includes both achieving homogenous ablation within the tumor (so no focal areas of viable tumor remain) and adequately treating a rim of apparently “normal” tissue around the tumor, which often contains microscopic invasion of malignant cells at the tumor periphery. Thus, based upon examinations of tumor progression in patients undergoing surgical resection and the demonstration of viable malignant cells beyond visible tumor boundaries, in most cases (or except when otherwise indicated) tumor ablation therapies attempt to include at least a 0.5- to 1-cm “ablative margin” of seemingly normal tissue for liver and lung, though less may be needed for some tumors in the kidney.6,7 A secondary goal is to ensure accuracy of therapy to minimize the damage to nontarget normal tissue surrounding the ablative zone. As such, one significant advantage of percutaneous ablative therapies over conventional standard surgical resection is the potential to remove or destroy only a minimal amount of normal tissue. This is critical in clinical situations where the functional state of the background organ parenchyma is as important in determining patient outcome as the primary tumor. Examples of clinical situations where this is relevant include focal hepatic tumors in patients with underlying cirrhosis and limited hepatic reserve, patients with von Hippel-Lindau syndrome who have limited renal function and require treatment of multiple renal tumors, and patients with primary lung tumors with extensive underlying emphysema and limited lung function.8–10 Many of these patients are not surgical candidates owing to limited native organ functional reserve, placing them at a higher risk for postoperative complications or organ failure. Other clinical circumstances in which high specificity and accuracy of targeting have proven useful include providing symptomatic relief for patients with symptomatic osseous metastases or hormonally active neuroendocrine tumors, and in using percutaneous therapies to improve focal interstitial drug delivery.2,11,12 Finally, maximizing ablation efficiency is an additional consideration. For example, appropriate and complete tumor destruction only occurs when the entire target tumor is exposed to appropriate temperatures, and therefore determined by the pattern of tissue heating in the target tumor. For larger tumors (usually defined as > 3-5 cm in diameter), a single ablation treatment may not be sufficient to entirely encompass the target volume.13 In these cases, multiple overlapping ablations or simultaneous use of multiple applicators may be required to successfully treat the entire tumor and achieve an ablative margin, though accurate targeting and probe placement can often be technically challenging.14 Tumor biology can also affect ablation efficiency. Growth patterns of the tumor itself can influence overall treatment outcomes, with slow-growing tumors more amenable to multiple treatment sessions over longer periods of time. Optimizing ablation efficiency is applicable to a wide range of ablative technologies, including both thermal and nonthermal strategies. Ablative tissue heating occurs though two specific mechanisms. First, an applicator placed within the center of the target tumor delivers energy that interacts with tissue to generate focal heat immediately around it. This approach is similar for all thermal ablation strategies regardless of the type of energy source used, though specific mechanisms of heat induction are energy specific (as discussed later in this chapter).1 The second mechanism of tissue heating in thermal ablation uses thermal tissue conduction.15 Heat generated around the electrode diffuses through the tumor and results in additional high-temperature heating separate from the direct energy/tissue interactions that occur around the electrode. The contribution of thermal conduction to overall tissue ablation is determined by several factors. Tissue heating patterns vary based upon the specific energy source used; for example, microwave-based systems induce tissue heating at a much faster rate than RF-based systems, so thermal conduction contributes less to overall tissue heating.15 Tumor and tissue characteristics also affect thermal conduction. As an example, primary hepatic tumors (hepatocellular carcinoma) transmit heat better than the surrounding cirrhotic hepatic parenchyma.13,16 Regardless of the energy source used, the endpoint of thermal ablation is adequate tissue heating so as to induce coagulative necrosis throughout the defined target area. Relatively mild increases in tissue temperature above baseline (40°C-42°C) can be tolerated by normal cellular homeostatic mechanisms.17 Low-temperature hyperthermia (42°C-45°C) results in reversible cellular injury, though this can increase cellular susceptibility to additional adjuvant therapies such as chemotherapy and radiation.18,19 Irreversible cellular injury occurs when cells are heated to 46°C to 48°C for 55 to 60 minutes and occurs more rapidly as the temperature rises, so most cell types die in a few minutes when heated at 50°C (Fig. 140-1).20 Immediate cellular damage centers on protein coagulation of cytosolic and mitochondrial enzymes and nucleic acid–histone protein complexes, which triggers cellular death over the course of several days.21 Heat fixation or coagulation necrosis is used to describe this thermal damage, even though ultimate manifestations of cell death may not fulfill strict histopathologic criteria of coagulative necrosis.22 This has implications with regard to clinical practice because percutaneous biopsy and standard histopathologic interpretation may not be a reliable measure of adequate ablation.22 Therefore, optimal temperatures for ablation range likely exceed 50°C. On the other end of the temperature spectrum, tissue vaporization occurs at temperatures above 110°C, which in turn limits further current deposition in RF-based systems (e.g., compared to microwave systems, which do not have this limitation). The exact temperature at which cell death occurs is multifactorial and tissue specific. Based on prior studies demonstrating that tissue coagulation can be induced by focal tissue heating to 50°C for 4 to 6 minutes,23 this has become the standard surrogate endpoint for thermal ablation therapies in both experimental studies and current clinical paradigms. However, studies have shown that depending on heating time, rate of heat increase, and the tissue being heated, maximum temperatures at the edge of ablation are variable. For example, maximum temperatures at the edge of the ablation zone, known as the critical temperature, have been shown to range from 30°C to 77°C for normal tissues and from 41°C to 64°C for tumor models (a 23°C difference).24,25 Likewise, the total amount of heat administered for a given time, known as the thermal dose, varies significantly between different tissues.24,25 Thus, the threshold target temperature of 50°C should be used only as a general guideline. This conceptual framework is also clearly applicable in determining optimal energy delivery paradigms for nonthermal energy sources.26 By far, the most well-studied and clinically ubiquitous and relevant percutaneous ablation source to date has been radiofrequency energy. During radiofrequency ablation (RFA), electric current from the generator oscillates between electrodes through ion channels present in most biological tissues. In this way, the RFA setup can be thought of as a simple electrical circuit where the current loop comprises a generator, cabling, electrodes, and tissue as the resistive element. Tissues are imperfect conductors of electricity (i.e., they have electrical impedance), so current flow leads to frictional agitation at the ionic level and heat generation, known as the Joule effect. Heating occurs most rapidly in areas of high current density; tissues nearest to an electrode are heated most effectively, whereas more peripheral areas receive heat by thermal conduction.27 Excessive ablative heating (to temperatures > 100°C) leads to tissue dehydration and water vaporization, which cause dramatic increases in circuit impedance, and ultimately limits further heating.28 When these effects begin to inhibit current flow from a generator, alternative methods (described later) to decrease circuit impedance, such as expanding the electrode surface area, pulsing the input power, and injections of saline, can be used to augment RF current flow.29,30 Microwaves are a second thermal energy source used for percutaneous image-guided tumor ablation. In contrast to RFA, where the inserted electrode functions as the active source, in microwave ablation (MWA) the inserted applicators (usually 14-17 gauge) function as antennae for externally applied energy at 1000 to 2450 MHz.31 The microwave energy applied to tissues results in rotation of polar molecules, which is opposed by frictional forces. As a result, there is conversion of rotational energy into heat.32 There are several potential advantages of MWA for tissue ablation.33,34 Microwaves readily penetrate through biological materials, including those with low electrical conductivity (e.g., lung, bone) and dehydrated or charred tissue. Consequently, microwave power can be continually applied to produce very high temperatures (>150°C), improving ablation efficacy by increasing thermal conduction into surrounding tissue.35 Microwaves also heat tissue more efficiently than RF energy in tissue, do not require ground pads, and multiple antennas can be operated simultaneously.36 The superposition of microwaves may even be exploited to augment performance.136–137 On the other hand, microwave energy is inherently more difficult to distribute than RF energy. Microwaves must be carried in waveguides (e.g., coaxial cable) that are typically more cumbersome than the small wires used to feed energy to RF electrodes, and prone to heating when carrying large amounts of power. It is well known that higher microwave powers increase ablation zone size, but excessive power in the antenna shaft can lead to unintended injuries to other tissues such as the skin.33,37 Recent investigations have shown that adding a cooling jacket around the antenna can reduce cable heating and eliminate skin burns while effectively increasing the amount of power that can safely be delivered to the tumor.38 In clinical practice, MWA has been utilized most in Japan and China, where several systems have been described.39,40 Most of these systems operate at 2.45 GHz and use monopole, dipole, or slotted coaxial antennas to deliver up to 60 W. Recently, 915 MHz and water-cooled systems have been described that appear to deliver up to 80 W and create larger ablations than previous generation systems.41 Recently a number of U.S. Food and Drug Administration (FDA)-approved MWA devices have become available for commercial use. Complete clinical characterization of each of these devices is currently ongoing. Laser ablation or laser photocoagulation is another method for inducing thermally mediated coagulation necrosis that has been employed for percutaneous tumor ablation. For this procedure, flexible thin optic fibers are inserted into the target through percutaneously placed needles, using imaging guidance. The laser provides sufficient energy to allow for significant heat deposition surrounding the fiber tip, inducing protein denaturation and cellular death. As with RF systems, thermal profiles have been demonstrated to correlate well with the extent of coagulation necrosis observed histopathologically,42,43 as well as with ultrasound43,44 and T1-weighted magnetic resonance (MR) images.45,46 Most devices use a standard laser source (Nd:YAG, erbium, holmium, etc.) that produces precise wavelengths. Additional device modifications have been developed to increase the size of the heated volume, including: (1) type of laser fiber (flexible/glass dome), (2) modifications to the tip (i.e., flexible diffuser tip or scattering dome), (3) length of applicator and diameter of the optic fiber, and (4) the number of laser applicators used (i.e., single vs. multiple applicators).47 Similar to RF technologies, additional device modifications such as pulsing algorithms and internal cooling of the applicator have also been reported.48 High-intensity focused ultrasound (HIFU) is a transcutaneous technique that has recently been studied for minimally invasive treatment of localized malignancy.49,50 HIFU uses a parabolic transducer to focus the ultrasound energy at a distance, creating a focused beam of energy with very high peak intensity. This focusing of energy has been likened to using a magnifying glass to focus sunlight.51 The focused energy is transmitted transcutaneously into the targeted tissue without requiring percutaneous insertion of an electrode or transducer. The ultrasound energy absorbed by tissue is converted to heat, which ablates tissue via coagulation necrosis. Since insertion of a percutaneous applicator is not required, HIFU can be considered the least invasive of the “minimally invasive” therapies. However, limitations in time and coverage, especially given respiratory motion, have limited most clinical investigations to benign conditions in static organs such as the breast,52 uterus,53 and prostate.54 Cryoablation induces focal tissue injury using alternating sessions of freezing and tissue thawing55–59 (Fig. 140-2). Minimally invasive cryoablation is performed using a closed specially constructed cryoprobe that is placed on or inside a tumor. In the past, cryoablation had to be performed in the setting of an open procedure owing to the large caliber of available probes; however, technologic developments have led to smaller probes that can be placed percutaneously under MR or computed tomography (CT) guidance. Liquid forms of inert gases are cycled through the hollow probes, resulting in tissue cooling. The two main types of systems use gas or liquid nitrogen, or argon gas. Many of the systems that use argon gas base their freezing on the Joule-Thompson effect.60–62 More recent work has involved probes that use “super-critical” nitrogen to cool faster and deeper into tissue.63 Cryoablation induces direct cellular injury through multiple mechanisms including tissue cooling.64 At low cooling rates, freezing primarily propagates extracellularly, which draws water from the cell and results in osmotic dehydration.65 The intracellular high solute concentration that develops leads to damaged enzymatic systems, protein damage, and injury to the cell membrane.66,67 At higher cooling rates, water is trapped within the cell, intracellular ice formation occurs, and organelle and membrane injury results.65,68 A second hypothesized mechanism of cryoablative tissue destruction centers on vascular injury consisting of mechanical injury to the vessel wall, direct injury to cells lining the vessel, and post-thaw injury from reperfusion.64 Mechanical injury to the vessel wall from intravascular ice formation results in increased vessel porosity, reduced plasma oncotic pressure, and perivascular edema.69 Endothelial injury further results from the exposure of underlying connective tissue to the vessel lumen and subsequent thrombus formation.70 Damage to endothelial cells occurs in a fashion similar to the direct cellular injury described earlier. Reperfusion injury results from the release of vasoactive factors after the tissue thaws, which leads to vasodilation and increased blood flow to treated areas. Subsequent high oxygen delivery71 and neutrophil migration72 into the damaged area results in increased free radical formation, and through the peroxidation of membrane lipids, further endothelial damage. The degree to which each mechanism of cellular and tissue injury described determines overall tissue destruction is governed by characteristics of the administration algorithm, including freezing and thawing rate, the minimum (i.e., coldest) temperature reached, and the duration that temperature was maintained.64 However, for current commonly used systems, argon provides a heat sink of about 9 kJ and can generate temperatures as low as −140°C inside the iceball, which expands by thermal conduction.73 Finally, the fact that the lethal isotherm (−20°C to −40°C) rests several millimeters inside the iceball boundary must be considered when using imaging to assess cryoablation treatment efficacy.74 Percutaneous irreversible electroporation (IRE) is new ablation technology and is most notable because it is presumed to be inherently nonthermal (i.e., no heat is produced to cause cell death). Rather, cells are eradicated using several microsecond- to millisecond-long pulses of electric current. The pulses generate electrical fields up to 3 kV/cm that cause irreversible damage to the cell membrane, thereby inducing apoptosis.75,76 Since IRE is predominantly nonthermal, heat sinks, such as large vessels, should have a much smaller influence on the ablation zone than with thermal treatments. IRE also appears to limit damage to more collagenous tissues and nerves, which if verified in larger trials will make it an attractive option for thermally sensitive areas (e.g., in the prostate, near large blood vessels, or the renal cortex).77 IRE electrodes consist of insulated 19-gauge (1.1 mm diameter) or larger needles with an exposed active portion of 1 to 4 cm. For most applications, multiple electrodes are required and spaced 1 to 3 cm apart to provide sufficient electrical field strengths for irreversible cell damage. A single-needle bipolar electrode is also available for more localized treatments. Initial studies required very high-voltage pulses, but recent reports have shown that lower-voltage pulses can be used when repeated several hundred times.78 Current IRE devices do have notable drawbacks, including generation of potentially dangerous electrical harmonics that can stimulate muscle contraction or cardiac arrhythmias. These techniques require general anesthesia and paralytic induction and have treatment times on the order of seconds that prevent treatment monitoring or adjustment. There is also a requirement for accurate placement of several needles to achieve moderate-sized ablations (≈3-4 cm), and a lack of coagulation around needle insertion sites, which theoretically could elevate bleeding complication risks. Ongoing research aims to minimize these complications. Applying high levels of energy in a pulsed manner, separated by periods of lower energy, is one such strategy that has been used with RF-based systems to increase the mean intensity of energy deposition.79 If a proper balance between high- and low-energy deposition is achieved, preferential tissue cooling occurs adjacent to the electrode during periods of minimal energy deposition, without significantly decreasing heating deeper in the tissue. Thus, even greater energy can be applied during periods of high-energy deposition, thereby enabling deeper heat penetration and greater tissue coagulation.80 Synergy between a combination of both internal cooling of the electrode and pulsing has resulted in even greater coagulation necrosis and tumor destruction than either method alone.80 Pulsed-energy techniques have also been successfully used for microwave and laser-based systems. Another strategy is to slowly increase (“ramp up”) the RF energy application in a continuous manner until the impedance to RF current flow increases prohibitively.61,81 This approach is often paired with multi-tined expandable electrodes, which have a greater contact surface area with tissue, and in which the goal is to achieve smaller ablation zones around multiple small electrode tines. This algorithm is also often combined with a staged expansion of the electrode system such that each small ablation occurs in a slightly different location within the tumor (with the overall goal being ablating the entire target region). Electrode switching is an additional technique incorporated into pulsing algorithms to further increase RF tissue heating.82 In this technique, multiple independently placed RF electrodes are connected to a single RF generator, and RF current is applied to a single electrode until an impedance spike is detected, at which point current application is applied to the next electrode, and so on. Several studies have demonstrated significant increases in ablation zone size and a reduction in application time using this technique.82,83 Brace et al. also show larger and more circular ablation zones with the switching application, with more rapid heating being 74% faster than sequential heating (12 vs. 46 minutes).82 Continued device development has also led to increases in the overall maximum amount of power that can be delivered.84,85 For RF-based devices, the maximum amount of RF current that can be delivered is dependent on both generator output and electrode surface area. Higher surface areas reduce the current density, and therefore adequate tissue heating around the electrode cannot be achieved. Initial systems had maximum power outputs of less than 200 W, but subsequent investigation suggests that higher current output and larger ablation zones can be achieved if higher-powered generators are coupled with larger surface area electrodes. For example, Solazzo et al. used a 500-KHz (1000 W) high-powered generator in an in vivo porcine model and achieved larger coagulation zones with a 4-cm tip cluster electrode (5.2 ± 0.8 cm) compared to a 2.5-cm cluster electrode (3.9 ± 0.3 cm).85 Similar gains in ablation size have been seen in higher-powered versions of microwave-based systems.86 Originally, simply lengthening the electrode tip increased coagulation in an asymmetric and preferentially longitudinal geometry. Use of a single electrode inserted multiple times to perform overlapping ablations requires significantly greater time and effort, making it impractical for routine use in a clinical setting. Therefore, using multiple electrodes simultaneously in a preset configuration represents a significant step forward in increasing overall ablation size. Initial work with multiple electrodes demonstrated that placement of several monopolar electrodes in a clustered arrangement (no more than 1.5 cm apart) with simultaneous RF application could increase coagulation volume by over 800% compared to a single electrode.87 Subsequently, working to overcome the technical challenges of multiprobe application, multi-tined expandable RF electrodes were developed.88 These systems involve deploying a varying number of multiple thin, curved tines in the shape of an umbrella (or more complex geometries) from a central cannula.89,90 This surmounts earlier difficulties by allowing easy placement of multiple probes to create large reproducible volumes of necrosis. Leveen et al., using a 12-hook array, were able to produce lesions measuring up to 3.5 cm in diameter in in vivo porcine liver by administering increasing amounts of RF energy from a 50 W RF generator for 10 minutes.91 More recently, Applebaum et al. were able to achieve over 5 cm of coagulation in in vivo porcine liver using currently commercially available expandable electrodes with optimized stepped-extension and power input algorithms.92
Image-Guided Thermal Tumor Ablation
Basic Science and Combination Therapies
Understanding Tumor Ablation: An Overview
Goals of Minimally Invasive Therapy
Principles of Tissue Heating in Thermal Ablation
Different Thermal Ablation Modalities
Radiofrequency
Microwave
Laser
Ultrasound
Cryoablation
Irreversible Electroporation
Advances in Radiofrequency Ablation: A Model for Technologic Evolution
Refinement of Energy Application Algorithms
Electrode Modification
Stay updated, free articles. Join our Telegram channel
Full access? Get Clinical Tree
Image-Guided Thermal Tumor Ablation: Basic Science and Combination Therapies
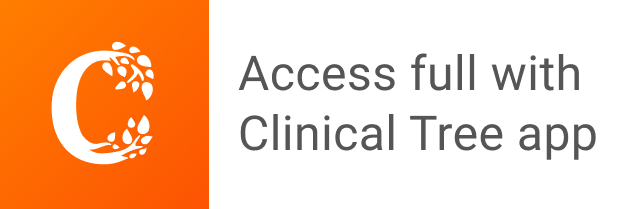