Peter Hoskin, Roberto Alonzi
Imaging for Radiotherapy Planning
Radiation therapy has been used as a treatment for cancer for more than 100 years, with its earliest roots dating back to the discovery of X-rays in 1895. Its development in the early 1900s is largely due to the work of Marie Curie (1867–1934), who discovered the radioactive elements polonium and radium in 1898. Despite these distant origins, radiotherapy remains at the forefront of the treatment for cancer. Approximately 60% of cancer patients currently receive radiation therapy at some stage during their illness with 75% of these treated with curative intent.1 Despite major advances in drug treatments for cancer, there has continued to be a steady annual increase in radiotherapy treatment that is unlikely to change within the next 10–20 years.2
As a result of advances in imaging and computer technology, radiotherapy has been transformed from 2-dimensional (2D) techniques to highly precise 3-dimensional (3D) conformal treatments that utilise axial tomographic images of the patient’s anatomy to guide 3D intensity-modulated, image-guided therapy. Advances in imaging have allowed radiation oncologists to delineate and target tumours more accurately, thereby producing better treatment outcomes, improved organ preservation and fewer side effects. Furthermore, with the development of functional imaging techniques such as positron emission tomography (PET), dynamic contrast-enhanced computed tomography (CT) and multi-parametric magnetic resonance imaging (MRI) it is now possible to integrate biological information (for example, tumour oxygenation, cellular proliferation or tumour blood flow) into the radiotherapy planning process. Imaging is therefore critical at almost every stage in the practice of modern radiation delivery (Fig. 73-1).
Types of Radiotherapy
External Beam Radiotherapy
External beam radiotherapy constitutes the mainstay of radiation treatment in developed countries. External beam radiotherapy refers to any situation where the source of the radiation is located at a distance from the patient and the beam of radiation is then directed towards a defined treatment area. Approximately 85% of all therapeutic radiation exposures are delivered using external beam techniques. Various types and energies of radiation can be delivered in this way, including electromagnetic radiation such as X-rays and γ-rays, or particles such as electrons and protons. Higher energies of radiation penetrate deeper into body tissues. As a result low-energy X-rays (60–300 keV) are reserved for the treatment of skin cancers and superficial subcutaneous tumours. Most external beam radiotherapy treatments utilise megavoltage X-rays or electrons (6–18 MeV) generated by a linear particle accelerator (Fig. 73-2).
Conventional External Beam Radiotherapy
Conventional radiotherapy refers to techniques in which the treatment volume is defined by simple geometric parameters. In general, no attempt is made to delineate the tumour outline or to shape the radiation dose distribution to conform to the tumour volume. This is commonly practised for palliative treatments where long-term normal tissue toxicity is less relevant. The irradiated volume can be defined clinically but more often fluoroscopic or CT simulation is used. The radiation fields tend to run parallel to each other, creating a box-like treatment volume (Fig. 73-3).
Three-Dimensional Conformal Radiotherapy
The incorporation of axial imaging data allows 3D reconstruction of the tumour and surrounding organs. This provides more accurate localisation of the target volume and more information regarding the amount of normal tissue that will be irradiated. The radiotherapy planning computer software then utilises the attenuation coefficient information (Hounsfield units) derived from the CT image on a voxel-by-voxel basis to predict the attenuation of each therapeutic radiation beam as it passes through the body. As a result, the number and profile of the radiation beams can be orientated and shaped to fit the profile of the target from a beam’s eye view using a multileaf collimator (Fig. 73-4). The resulting radiotherapy plan can be displayed as a colour map of radiation dose overlaid onto the anatomical CT images so that the radiation oncologist can determine whether the tumour volume will receive sufficient irradiation with acceptable normal tissue dose sparing (Fig. 73-5). By reducing the irradiated volume and dose to the sensitive surrounding normal tissues, this technique facilitates the delivery of higher tumour radiation doses than would be achievable using conventional techniques.
Intensity-Modulated Radiotherapy (IMRT)
IMRT represents a further step in the development of high-precision radiotherapy delivery. The term refers to a variety of techniques in which the radiation beams are not only shaped and orientated to conform to the tumour volume but also the intensity of radiation is modulated across each treatment beam. This technique can produce dose distributions that conform highly to complex shapes, including treatment volumes that wrap around sensitive normal structures such as the spinal cord (Fig. 73-6), enabling high-dose delivery to the tumour volume whilst sparing dose to the normal structures.
IMRT can be achieved using a number of different technologies. Most commonly, several static radiation fields in the same plane of orientation are used, similar to the situation for 3D conformal radiotherapy, but with varying dose flux across the profile of the beam which is achieved by moving the leaves of the multileaf collimator across the beam at varying rates.3 Alternatively, arc therapies utilise a number of non-coplanar beam arcs in which the radiation is delivered using multiple ‘stop and shoot’ beams or as a continuously moving field that varies in intensity throughout rotation.4 Tomotherapy is another technique for achieving IMRT in which a megavoltage X-ray source is mounted in a similar fashion to a CT X-ray source (Fig. 73-7). The treatment volume is irradiated using the machine’s continuously rotating beam that is modulated in intensity whilst the patient moves through the gantry bore.5
Stereotactic Body Radiotherapy (SBRT)
In SBRT the distribution of radiation beams is in three dimensions and not in two as in traditional radiotherapy.6 It is based on a precise evaluation of the positioning of the tumour in real time and on the delivery of the dose by multiple beams (sometimes one hundred or more beams per treatment—Fig. 73-8). The treatment is based upon stereotactic radiosurgery methods for the treatment of intracranial tumours. However, it can only be used for selected, small extracranial lesions such as tumours of the lung and prostate or small solitary metastases. Even with small tumours, the complexity of the technique can sometimes result in treatment times of over an hour per fraction, thereby necessitating a small number of radiotherapy fractions to make a course of SBRT treatment clinically (and economically) feasible. Fortunately, the precision of SBRT allows high doses to be delivered in a very limited number of fractions. This is sometimes referred to as ultra-hypofractionation in which doses per fraction can be as high as 20 grays (conventional radiotherapy is delivered at 2 grays per fraction). There is current speculation that the mechanism of radiation-induced tumour cell killing may differ at such high doses per fraction. It has been hypothesised that radiation doses of more than 10 grays per fraction can cause rapid obliteration of the tumour vasculature, which cannot be achieved with the lower conventional doses, resulting in a biological advantage for ultra-hypofractionation.
Brachytherapy
Brachytherapy refers to a situation in which a radioisotope is placed onto or inside the patient. The radiation source is sealed in a protective capsule or wire which prevents the radioisotope from moving or dissolving in body fluids but allows the emission of ionising radiation (in the form of α, β, γ or X-radiation) to the surrounding tissues. The source can be placed into the target tissues or tumour itself such as the prostate or breast (interstitial brachytherapy), into a body cavity such as the uterine cavity, oesophagus or bronchus (intracavitary/intraluminal brachytherapy) or onto the skin surface to treat a cutaneous malignancy.
In certain situations brachytherapy offers some major advantages over external beam techniques. One of the key features of brachytherapy is that the irradiation only affects a very localised area around the radiation sources as dose falls off rapidly, obeying the inverse square law. As long as the sources are precisely placed within the tumour, there is minimal exposure to radiation of healthy tissues further away from the sources. This allows very high doses to be administered to the target volume (Fig. 73-9). Also, patient set-up and tumour motion are less relevant because the radiation sources move with the tumour and therefore retain their correct position; this increases the confidence that the radiation has been delivered in accordance with the required plan.
Due to the very short distances between the radiation source and the treatment volume, the absorbed tissue dose is almost entirely a function of the distance from the source, rather than due to the attenuation coefficient of the tissues that the radiation passes through (in contrast to the situation with external radiation). Radiation from a point source is inversely proportional to the square of the distance from the source. Tissues twice as far away receive only one-quarter of the dose in the same time period. As a result, brachytherapy techniques do not necessarily require Hounsfield number information from CT data to produce conformal treatment plans. As long as the position of the radiation sources can be reliably located, any imaging modality can be used for planning (Fig. 73-10).
Brachytherapy applicators have been adapted to enable better imaging. For example, CT- and MRI-compatible intrauterine tubes have been developed for gynaecological treatments. Seeds containing 125I are commonly used to treat prostate cancer and have been designed with roughened and bevelled ends to maximise ultrasound visibility. Because precise reproduction of the source position is critical to brachytherapy dose calculations, any distortion or registration error may be critical and should be carefully quality-assured before incorporating in routine practice. Specific MR sequences may be developed to enable certain applications: for example, proton-rich sequences for identification of 125I seeds in the prostate.7 Imaging also has a crucial role in the accurate positioning of radiation sources or afterloading applicators for brachytherapy. Its flexibility and real-time imaging capability means that ultrasound is considered particularly valuable in this respect.
Brachytherapy is often defined by the rate at which the radiation dose is applied. Low-dose rate (LDR) brachytherapy sources emit radiation at a rate of up to 2 Gy⋅h−1. These sources can be permanent, as is the case with LDR prostate brachytherapy (Fig. 73-11), or removed after several days, as for oral cavity tumours. High-dose rate (HDR) brachytherapy sources emit radiation at a rate of over 12 Gy⋅h−1; however, the most commonly used HDR units with an 192Ir source emit radiation at a much higher rate of between 60 and 100 Gy⋅h−1. HDR sources are always afterloaded (instead of directly placing the source into the patient); hollow catheters are inserted and subsequently connected to the HDR unit which can be operated remotely to deliver the source into each catheter with the operator outside the shielded room (Fig. 73-12).
Particle Therapy
Also known as hadron therapy, particle therapy is an external beam technique in which highly energetic particles such as protons, neutrons or positive ions are directed at the tumour. Although electrons are particles, these are not usually considered in the category of particle therapy. The most common form of particle therapy uses protons that are accelerated by a cyclotron or synchrotron. Protons have several physical characteristics that provide an advantage over photon treatments. Protons penetrate deep into body tissues and deposit the majority of their energy in the last few millimetres of their range with virtually no radiation passing beyond this distance.8 Therefore, varying the energy of the proton beam can control the depth of treatment.
For most treatments, protons of different energies are applied to treat the entire tumour. Also, due to their greater mass, protons scatter less and the beam remains focused with less broadening than photon beams. These properties translate into a clinical advantage when it is paramount to limit the radiation dose to critical normal structures that lie deep to the tumour. In particular, where there may be a critical normal tissue such as the spinal cord immediately behind the target, the minimal exit dose using proton therapy can prevent major long-term morbidity. This is particularly true for paediatric neoplasms such as medulloblastoma where there is convincing clinical data to show the advantage of sparing the developing brain and cord.9–11
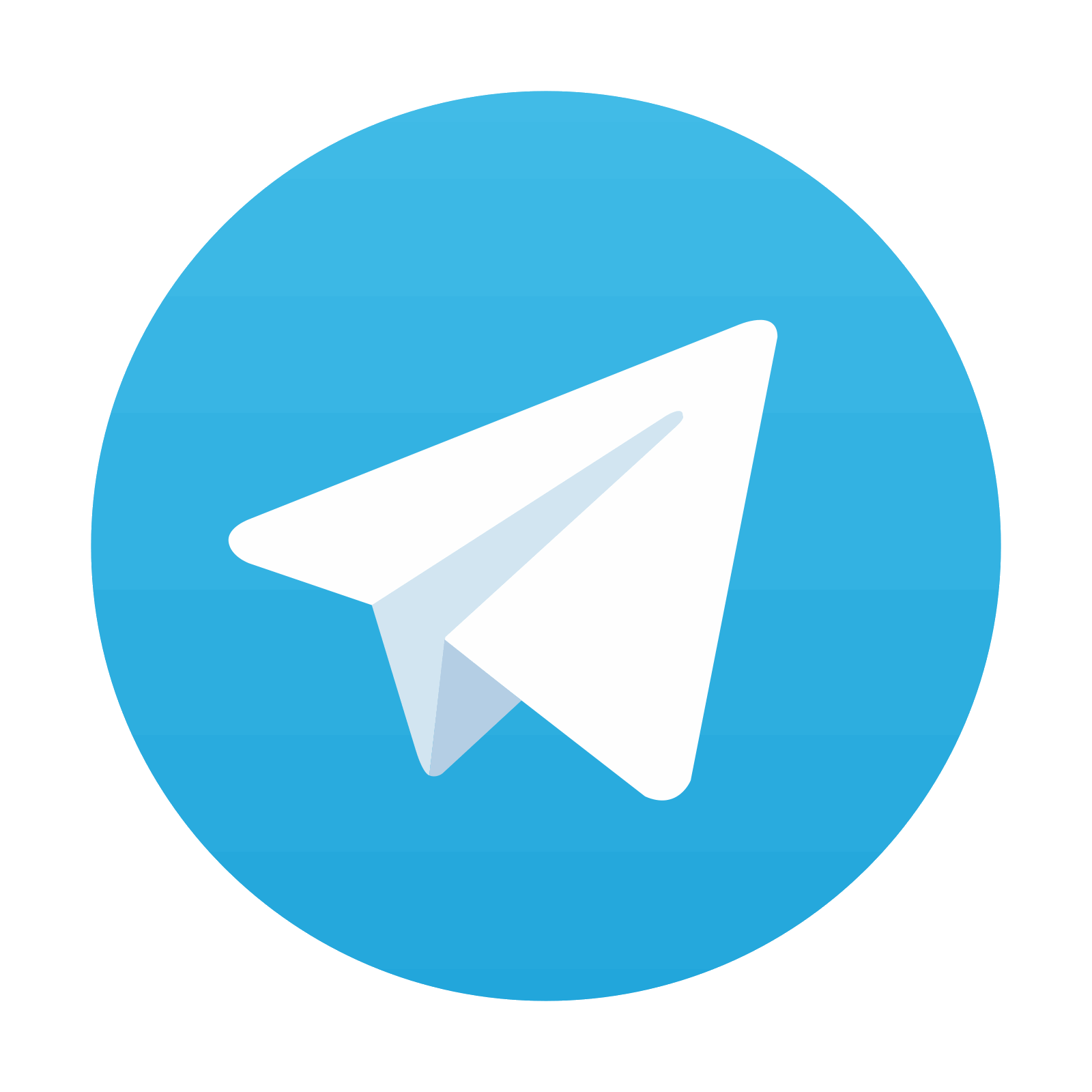
Stay updated, free articles. Join our Telegram channel
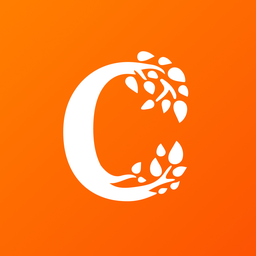
Full access? Get Clinical Tree
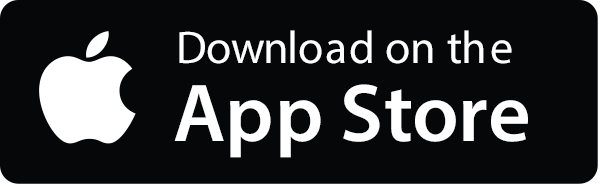
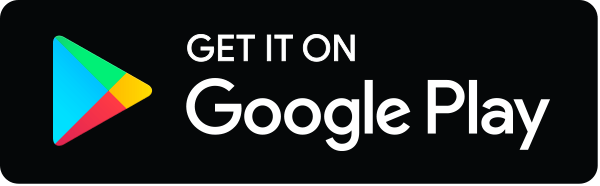