Imaging the Neovasculature
A. J. Beer
H. J. Wester
M. Schwaiger
Angiogenesis, the formation of new blood vessels, is a fundamental process involved in a variety of physiological as well as pathological conditions. Physiologically, it is required for development, wound repair, reproduction, and response to ischemia. Pathologically, it is associated with disease conditions like arthritis, psoriasis, retinopathies, and cancer (1). Especially in oncology, angiogenesis is an essential process for tumor growth and tumor metastasis, since the growth of solid tumors remains restricted to 2 to 3 mm in diameter until supported by the onset of angiogenesis. This concept was first proposed by Folkman (2) in 1971 and initiated intense research efforts and detailed investigations on the biology of angiogenesis, which subsequently have identified more than 20 angiogenic growth factors, their receptors, and signal transduction pathways.
Endogenous angiogenesis inhibitors have been discovered, and the cellular and molecular characterization of the angiogenic phenotype in human cancers has been achieved (2,3,4). Over the past few years, the concept of antiangiogenic therapy has evolved as a therapeutic strategy for diseases associated with increased angiogenic activity, like arthritis, psoriasis, and especially malignant neoplasms. In clinical oncology, antiangiogenic therapy is aimed at stopping cancer progression by suppressing the tumor blood supply (1).
Although the results of the first clinical trials using angiogenesis inhibitors did not meet the then very high expectations, encouraging results have recently been achieved with the anti–vascular endothelial growth factor (VEGF) antibody bevacizumab (Avastin) in combination with standard cytotoxic chemotherapy in metastatic colorectal cancer, breast cancer, and non–small cell lung cancers (5,6,7,8). In part, the disappointing results of the first antiangiogenic agents might be due to the difficulties in designing clinical trials for such drugs, which, unlike traditional chemotherapeutic agents, are not cytotoxic and usually have limited side effects. The concept of looking for the maximum tolerated dose is potentially not appropriate for most antiangiogenic drugs. Another problem in trial design is defining tumor response with these new agents, which are often not primarily cytotoxic in their mechanisms of action.
Clinical trials with conventional cytotoxic chemotherapeutic agents usually use morphological imaging such as computed tomography (CT) or magnetic resonance imaging (MRI) to provide indices of therapeutic response. Linear measurements in one or two dimensions are mainly used to estimate changes in tumor size in response to the investigational therapy as compared with a baseline measure. The introduction of the RECIST (Response Evaluation Criteria for Solid Tumors) criteria in 2000 resulted in considerable progress in standardizing these measurements (9). However, since antiangiogenic agents often lead to a decrease of tumor progression rather than to tumor shrinkage, the assessment of tumor response by conventional imaging methods might take months or years. New biomarkers of early tumor response to noncytotoxic drugs, which predict subsequent clinical response, are badly needed (10). Such biomarkers would not only facilitate clinical trials of new drugs but could also be used to aid in the selection of optimal treatment for individual patients (“personalized medicine”).
There is great interest in imaging techniques that can be used as biomarkers and will provide an early indicator or predictor of effectiveness at a functional or molecular level. Currently, changes in hemodynamic parameters such as blood flow, blood volume, or vessel permeability are evaluated as biomarkers for response evaluation in trials with antiangiogenic drugs. Various imaging techniques are used for this purpose, mainly dynamic contrast enhanced MRI (DCE MRI), but also positron emission tomography (PET) (mostly with oxygen-15 [15O]-water), dynamic contrast enhanced CT, and ultrasound (11). For all these techniques, significant correlations with conventional markers of angiogenesis, like microvessel density (MVD) or VEGF expression in immunohistochemistry, have been demonstrated, although the results of different studies are far from being uniform as these techniques vary substantially in their methods from center to center (12,13,14,15,16,17).
All the techniques mentioned above have their inherent technical problems that limit their general use in clinical routine and make the results difficult to interpret. Although PET with [15O]-water is a truly quantitative method that has been quite comprehensively evaluated with good results for perfusion measurements of the heart and brain, the results in malignant tumors are harder to interpret (18). The reason for this is that vessels in tumors are leaky,
tortuous, and have dead ends as well as shunts between the arterial and venous systems. Tumors often have relatively low blood flow, causing a low and variable signal from the tumors. Moreover, the production of [15O]-water with its short half-life requires a cyclotron, which limits the use of this technique to a few PET centers. DCE MRI on the other hand is widely available and uses U.S. Food and Drug Administration–approved contrast agents with reasonably low toxicity like Gd-DTPA (gadolinium diethylenetriamine penta-acetic acid; e.g., Magnevist). However, MRI has several challenges regarding quantitation, and changes in the design of the pulse sequences influence the results and make them difficult to compare between institutions and across different manufacturers of MRI equipment (19).
tortuous, and have dead ends as well as shunts between the arterial and venous systems. Tumors often have relatively low blood flow, causing a low and variable signal from the tumors. Moreover, the production of [15O]-water with its short half-life requires a cyclotron, which limits the use of this technique to a few PET centers. DCE MRI on the other hand is widely available and uses U.S. Food and Drug Administration–approved contrast agents with reasonably low toxicity like Gd-DTPA (gadolinium diethylenetriamine penta-acetic acid; e.g., Magnevist). However, MRI has several challenges regarding quantitation, and changes in the design of the pulse sequences influence the results and make them difficult to compare between institutions and across different manufacturers of MRI equipment (19).
Until recently, these techniques have shown mixed results. Some studies show no effect at all with DCE MRI in response to therapy. In a phase I study, DCE MRI was used to evaluate the effects of the VEGFR2 inhibitor SU5416 in patients with treatment refractory solid tumors, including soft tissue sarcoma, melanoma, renal cancers, and other entities. No changes in the hemodynamic parameters Ktrans (rate transfer constant) and γe (volume of the extravascular extracellular space per volume of tissue) were seen in response to treatment (20). Other studies successfully showed the effects of therapy, but no correlation with the response to treatment.
In patients with inflammatory and locally advanced breast cancer treated with bevacizumab alone for one cycle and subsequently in combination with chemotherapy and examined with DCE MRI at baseline and after cycles one, four, and seven, all hemodynamic parameters showed significant decreases after treatment with bevacizumab alone, and continued to decrease after the start of chemotherapy. However, there was no significant correlation of any of these parameters with clinical response (21). On the other hand, there are also promising results that show a correlation between response to treatment and the results of DCE MRI. One study evaluated DCE MRI in patients with colorectal cancer and metastatic liver lesions who received the VEGF receptor tyrosine kinase inhibitor PT787/ZK222584. Twenty-six patients were examined at baseline and one or more time points during treatment. A significant negative correlation between the DCE MRI pharmacokinetic parameter examined (Ki, which is related to Ktrans) and both the oral dose and plasma levels of PT787/ZK222584 were found. Correct response evaluation was possible, as significantly greater reductions in Ki were found for responders with complete remission, partial remission, or stable disease according to the RECIST criteria than for nonresponders (22).
These different results emphasize that the role of DCE MRI for response evaluation is complex and still has to be further evaluated in order to define its value as a surrogate end point. In the future, markers at the molecular level might be used for response evaluation of antiangiogenic agents, which hopefully will provide more specific information compared to the functional assessment of hemodynamic parameters.
PET tracers for assessment of glucose metabolism or proliferation like fluorine-18-fluorodeoxyglucose ([18F]-FDG) and fluorine-18-fluorothymidine ([18F]-FLT) have already shown promising results in clinical studies for response assessment of cytotoxic chemotherapies (23,24). In the same way, targeting specific molecular markers of angiogenesis might be used for response assessment of antiangiogenic therapies, like the VEGF pathway or cell surface markers like the integrin αvβ3.
The Biology of Angiogenesis
Angiogenesis is a very complex process involving a multitude of growth factors, cell surface receptors, enzymes, and so forth. A basic knowledge of the biology of angiogenesis is mandatory for a thorough understanding of the different approaches used for imaging the neovasculature with PET. The cascade of angiogenesis will be discussed in the following section. A detailed review of the processes of angiogenesis can be found elsewhere (25,26), on which the following explanations are based.
The same applies to the different antiangiogenic therapeutic strategies, which are currently being evaluated, because knowing the most promising targets and their ligands for antiangiogenic therapy facilitates understanding of the most common strategies used for imaging these targets with PET (27,28).
The Cascade of Angiogenesis
The lack of access to circulating oxygen, growth factors, and nutrients in early tumor development limits tumor growth because tumors do not have their own blood supply. Consequently, solid tumors initially are composed of only a small population of transformed cells whose growth is controlled by a balance between apoptosis and tumor cell proliferation. To overcome this problem, tumors grow toward pre-existing nearby blood vessels. Tumor cells may then infiltrate these blood vessels regionally and form vessels consisting of normal endothelial cells mixed with infiltrative tumor cells called a “mosaic vessel” (29). Since this process mainly serves the tumor periphery, further tumor expansion leads to increasing central hypoxia. This initial phase of limited tumor growth may persist for months or even years.
The next step in the process of angiogenesis is called the angiogenic switch, because the tumor switches to its angiogenic phenotype. It is a phase of rapid tumor growth and involves a multitude of peptide angiogenic factors that are produced in response to tumor hypoxia. These include the VEGF, the acidic and basic fibroblast growth factors (aFGF, bFGF), and platelet-derived endothelial cell growth factor (PD-ECGF) (30). Local angiogenesis inhibitors like thrombospondin-1, endostatin, angiostatin, or antiangiogenic antithrombin III can also be found in tumor tissue.
The angiogenic switch occurs when the tumors produce angiogenic factors in excess of local angiogenesis inhibitors. The angiogenic growth factors diffuse toward nearby pre-existing blood vessels and bind to receptors located on endothelial cells like receptors to VEGF (VEGF-R1/Flt-1, VEGF-R2/KDR/Flk-1, VEGF-R3/KDR, Flt-1, VEGF-R2, VEGF-R3/Flt-4, VEGF-R4/neuropilin-1) (31). The binding of ligands to their receptors leads to activation of endothelial cells by receptor dimerization and activation of various signal transduction pathways, like phosphorylation of tyrosine kinases, protein kinases, and mitogen-activated protein kinases (32,33,34,35,36,37,38). Once endothelial cells become activated, the original vessels undergo characteristic morphological changes and form mother vessels, which are characterized by basement membrane degradation, a thinned endothelial cell lining, increased endothelial number, decreased pericyte numbers, and pericyte detachment (39). These vessels have an enlarged diameter and are hyperpermeable compared to normal microvessels (40). Consequently, the earliest histopathological features of angiogenesis are microvascular dilatation, hyperpermeability, edema, and extravascular fibrin deposition. This transient process only lasts for a few days and in the next
step, mother vessels undergo at least four divergent morphological transformations (40,41,42,43).
step, mother vessels undergo at least four divergent morphological transformations (40,41,42,43).
The first mechanism for tumor vascularization is sprouting angiogenesis, which involves the proliferation and migration of endothelial cells from pre-existing blood vessels and the organization of tubular vascular structures (44). This is the most important and best understood process, and it requires the focal dissolution of the basement membrane of surrounding mother vessels. This is achieved by a number of proteolytic enzymes, like matrix metalloproteinases and plasminogen activator, which enable endothelial cells to exit the vessel abluminally (45).
Activated angiogenic endothelial cells proliferate rapidly and migrate into the extracellular matrix toward the angiogenic stimulus (46,47). Important factors in this step of angiogenesis are adhesion molecules known as integrins, such as the αvβ3 and αvβ5 integrin, which facilitate migration and vascular survival (48,49,50,51). Collagenases such as matrix metalloproteinases are secreted at the sprouting tips of growing vessels by endothelial cells and are responsible for the degradation of the extracellular matrix and facilitate cell invasion (52,53).
Finally, a lumen within an endothelial cell tubule has to be formed to allow for the supply of nutrients and oxygen to the tumor via circulation. This requires interactions between the extracellular matrix and cell-associated surface proteins, such as galactin-2, platelet endothelial cell adhesion molecule-1 (PECAM-1), and VE-cadherin (54,55,56). The newly formed vessels are stabilized through the recruitment of smooth muscle cells and pericytes. In this process, the angiopoietin family plays a major role, like angiopoietin-1 (Ang-1), which binds to the Tie-2 receptor on angiogenic endothelium (49).
Besides sprouting angiogenesis, various other processes may occur. Mother vessels may retain their large diameter and evolve into medium-sized arteries and veins by acquiring a smooth muscle and internal elastica. This process takes from a few days to several months. Alternatively, the endothelium of a mother vessel may form smaller separate well-differentiated vessel channels by projecting cytoplasmic structures into the lumen, which form transluminal bridges. This takes from several days to 3 weeks.
Another process is called intussusception, which involves focal invagination of connective tissue pillars from within the mother vessel and takes from several days to several weeks (41,42). During intussusception, two opposite endothelial cell membranes get in contact with each other and interendothelial junctions develop. The gap between the two newly formed vessels is filled by mesenchymal cells composed of fibroblasts and pericytes to form a pillar or an interstitial or intervascular tissue structure, and extracellular matrix proteins, such as collagen or fibrin, accumulate within the pillar. Intussusception generates vessels more rapidly than sprouting. The molecular mechanism of intussusception is not fully understood, but an increasing rate of blood flow plays a major role, as well as shear stress, which can be sensed by endothelial cells and transduced inside the cell by PECAM-1, resulting in increased expression of angiogenic factors and adhesion molecules.
Antiangiogenic Therapeutic Strategies
Antiangiogenic drugs are classified according to their main mechanism of action. True angiogenesis inhibitors do not destroy pre-existing blood vessels within a tumor, but only stop the formation of neovasculature. The expected effect of true angiogenesis inhibitors is thus disease stabilization rather than tumor regression. Contrary to that, vascular targeting agents also destroy the pre-existing tumor vasculature. Finally, nonselective antiangiogenic agents, which are basically conventional chemotherapeutic agents, show cytotoxic, antiproliferative, or anti-invasive effects on multiple cell types, including angiogenic endothelial cells. Especially at low concentrations, several conventional cytotoxic chemotherapeutic drugs have shown antiangiogenic effects. A multitude of antiangiogenic drugs targeting different steps in the angiogenic cascade have been or are currently being studied in clinical trials, and only a short overview of the most important ones are presented here.
Growth Factor Antagonists and Endothelial Cell Signal Transduction Inhibitors
Several drugs, such as suramin, interferon-α, and Angiozyme (Ribozyme Pharmaceuticals, Boulder, Colorado), suppress production of angiogenic growth factors. Monoclonal antibodies and soluble receptors have been developed against VEGF, with bevacizumab being the most promising agent in this group. Bevacizumab showed encouraging results in metastasized colorectal cancer, breast cancer, and non–small cell lung cancer (5,6). Small molecule drugs that inhibit the endothelial signal transduction caused by specific growth factor-receptor binding are currently being tested in clinical trials. Most of them are tyrosine kinase inhibitors. Both selective (against VEGF or PDGF) and nonselective agents are under evaluation. Two of these nonselective small molecule tyrosine kinase inhibitors, SU11248 (sunitinib [Sutent]) and BAY-43-9006 (sorafenib [Nexavar]) have shown antitumor activity in clinical trials in patients with gastrointestinal stromal tumor refractory to imatinib (Gleevec) and in metastasized renal cell cancer. Due to these encouraging results, they have recently been approved as monotherapy for kidney cancer (5,29).
Inhibitors of Endothelial Cell Proliferation
A variety of antiangiogenic agents, such as TNP-470, thalidomide, squalamine, and captopril, inhibit endothelial cell proliferation. Treatment with TNP-470 has been shown to be more effective in limiting the growth of micrometastases than the growth of established tumors. It has shown some stabilization of disease in clinical trials of patients with Kaposi sarcoma and cervical cancer (28).
Inhibitors of Integrin Activation
Integrins are heterodimeric transmembrane glycoproteins that play an important role in cell–cell and cell–matrix interactions. Among them, the subtypes αvβ3 and αvβ5 have been well examined and are expressed on angiogenic endothelial cells and on some metastatic tumor cells, but not on quiescent endothelium. Blocking the αvβ3 integrin by monoclonal antibodies or cyclic peptides can lead to activation of p53 and endothelial cell apoptosis. Examples of drugs in clinical trial include Vitaxin (MedImmune, Gaithersburg, Maryland; humanized antibody to αvβ3 LM-609) and EMD-121974 (Cilengitide, Merck, Darmstadt), a cyclic pentapeptide with highly specific binding to αvβ3 and αvβ5. These agents are currently being evaluated in phase I through III trials comprised of patients with irinotecan refractory colorectal cancer, patients with glioblastoma in combination with temozolomide, and patients with Kaposi sarcoma, among other trials.
Matrix Metalloproteinase Inhibitors
Matrix metalloproteinases (MMP) are interesting targets for antiangiogenic treatment because their inhibition interferes with both endothelial and tumor cell invasion into the extracellular matrix at primary and metastatic sites. The gelatinases MMP-2 and MMP-9 are closely associated with angiogenesis and are the most promising targets among the family of MMPs, which consists of at least 20 distinct enzymes. Selective and nonselective MMP inhibitors, which include Marimastat (British Biotech, Inc, Oxford, United Kingdom), AG-3340 (Prinomastat, Agouron Pharmaceuticals, Pfizer, San Diego, California), Col-3, Neovastat (Aeterna Laboratories, Quebec City, Canada), and BMS-275291, are in advanced clinical trials. Marimastat demonstrated a survival benefit in patients with metastatic gastric cancer and in patients with glioblastoma treated in combination with temozolomide. However, it is also one of the first antiangiogenic agents that demonstrated dose-limiting side effects, mostly severe inflammatory polyarthritis (28).
Imaging of Functional Markers of Angiogenesis
Imaging of functional hemodynamic parameters like blood flow and blood volume with PET are not new techniques. Such methods have been widely applied and thoroughly evaluated in brain and heart tissue. Although there is some experience with using these techniques for assessment of functional parameters in tumors, their use in this respect is still limited and warrants further evaluation. For a good overview of this topic, see the excellent review of Laking and Price (18).
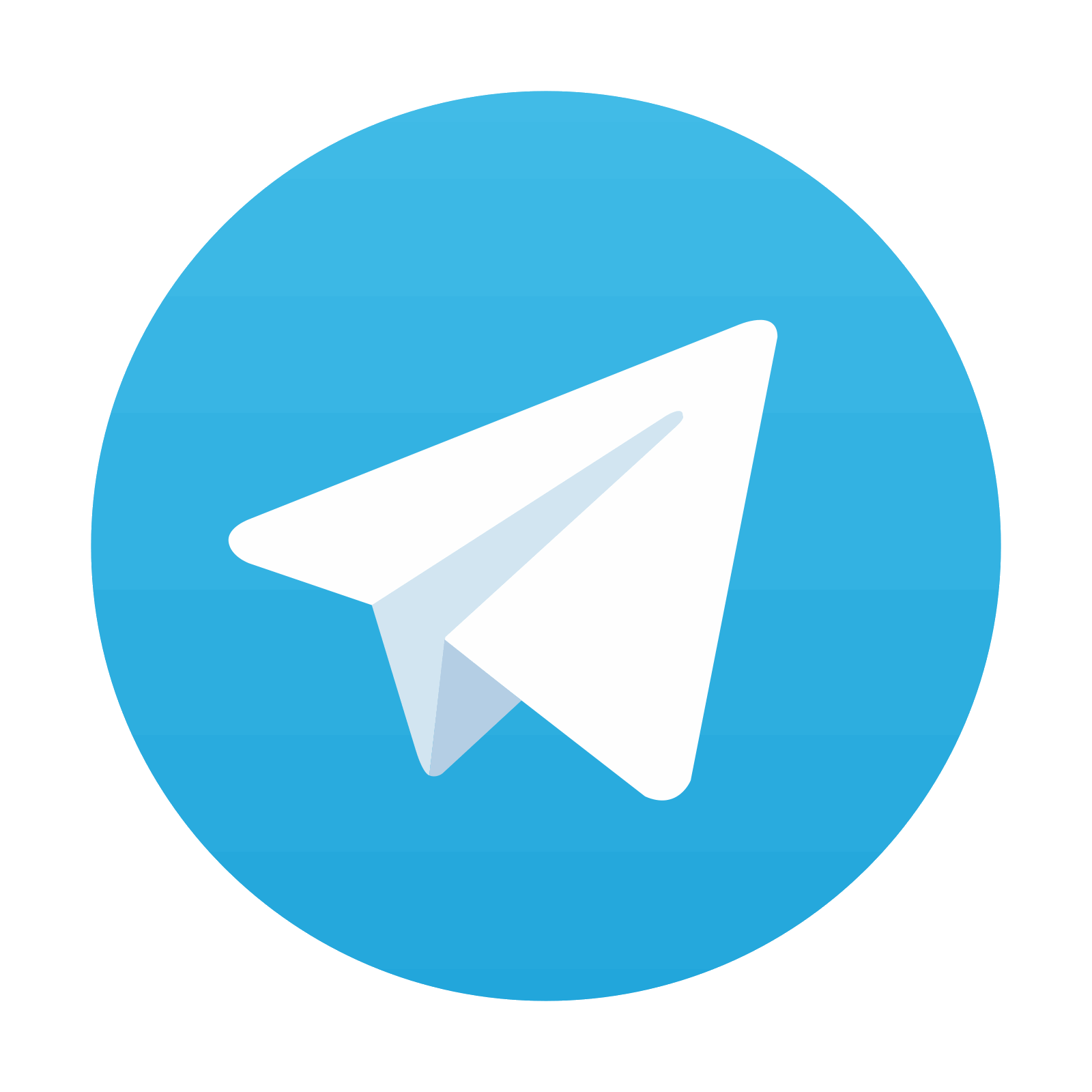
Stay updated, free articles. Join our Telegram channel
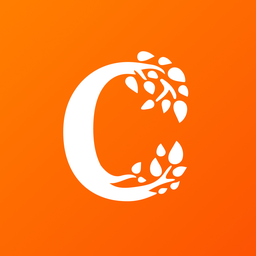
Full access? Get Clinical Tree
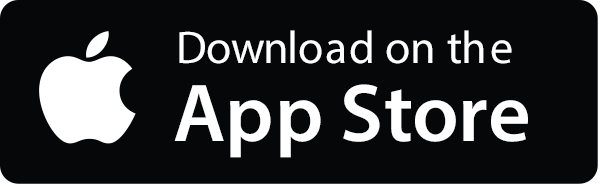
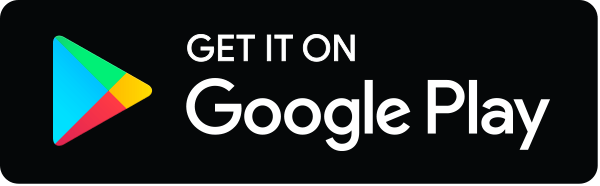