Fig. 1
A typical plan used for intraoperative radiation therapy using high-dose-rate brachytherapy (HDR-IORT). Dose distribution of an IORT plan prescribing 10 Gy to the surface in the (a) sagittal (b) coronal, and (c) axial planes. In (d), we see the catheters are placed in a silicone Freiburg flap that can be cut to conform to the tumor bed. After the wires are secure, the flap is placed into the operative field with the appropriate shielding. A wire with a single high-activity source (typically iridium-192) at the end then passes through the catheters imbedded into the flap at evenly spaced 1 cm intervals to deliver the radiation
Next, a single high-activity source, typically iridium-192, is programmed to “dwell” at prespecified locations in each needle or catheter as per the radiation treatment plan. The high activity of the source results in treatment times of minutes in contrast to hours or days, typical of LDR brachytherapy. Another advantage is the use of remote afterloading to guide the radioactive source from its shielded container through transfer tubes, to the treatment applicators implanted in the patient. This results in no radiation exposure to staff or caregivers as treatment can be given inside a shielded suite with the patient being remotely monitored with real-time video, audio, and even cardiac telemetry.
The following sections will detail specific applications of brachytherapy in the management of patients with hepatobiliary malignancy in terms of methods and treatment results.
2 Intraluminal Biliary Brachytherapy
The presence of malignant biliary obstruction, either from a primary hepatobiliary tumor or from secondary metastatic disease, often requires the placement of an intraluminal catheter to promote bile drainage and for symptom relief. Once in place, these catheters can serve as a conduit to load brachytherapy sources for palliation of unresectable tumors, to boost the external beam dose, or as a preoperative approach prior to hepatic transplantation in potentially curative tumors [6–12].
2.1 Methods
Intraluminal brachytherapy for hepatobiliary tumors requires a multimodality approach including both interventional radiology and radiation oncology. The interventional radiologist first places a needle using a percutaneous approach into the liver, finding the dilated bile duct using real-time image guidance including CT or fluoroscopy. Next, the location and extent of the tumor is characterized by a transhepatic cholangiogram (THC). Following this, a thin guide wire is threaded through the percutaneous needle, passed the obstructing tumor and into the duodenum. A flexible catheter is advanced over the guide wire and into the duodenum. If the catheter cannot be passed due to obstruction, it can be left in place to drain externally. After several days of drainage, it is often possible to advance the catheter distal to the tumor and into the duodenum due to decreased tissue edema. If the catheter can be passed by any portion of the tumor, brachytherapy may be useful to facilitate passage past the obstruction [12] Once the catheter is in place, it can be used for both internal and external biliary drainage [13–15].
For brachytherapy treatment, a Tuohy-Borst sidearm adapter is fixed to the external portion of the catheter. This two-arm adapter allows both external biliary drainage and delivery of brachytherapy. Following this, a blind-ended brachytherapy nylon treatment catheter with stainless steel wire (to prevent kinking) is inserted through the Tuohy port into the larger diameter drainage catheter and is advanced into position under fluoroscopy.
It is important to account for the radioactive source diameter when selecting catheter size, as the catheter must have room to accommodate the radioactive source. Typically, LDR sources require an 8–10 French catheter; HDR sources require an 8–14 French catheter, depending on the type of HDR source and catheter used. The Varisource® afterloader has a narrow source; the Gamma-Med® and Nucletron® afterloaders have a wider diameter source. It is good practice to insert the afterloading catheter into the drainage catheter and ensure easy passage before the performing the procedure.
The stainless steel wire is replaced with a dummy wire containing radiopaque markers at 1-cm intervals. An orthogonal radiograph or CT scan is obtained for brachytherapy planning purposes (Fig. 2). To deliver the brachytherapy treatment, the catheter is connected to an afterloader (for HDR brachytherapy) or an iridium or radium wire is inserted (for LDR brachytherapy). Once the prescription dose of radiotherapy has been delivered, the source is retracted and the drainage catheter flushed. It is recommended to keep a permanent biliary stent in place for 4–6 weeks to prevent biliary stenosis or stricture.
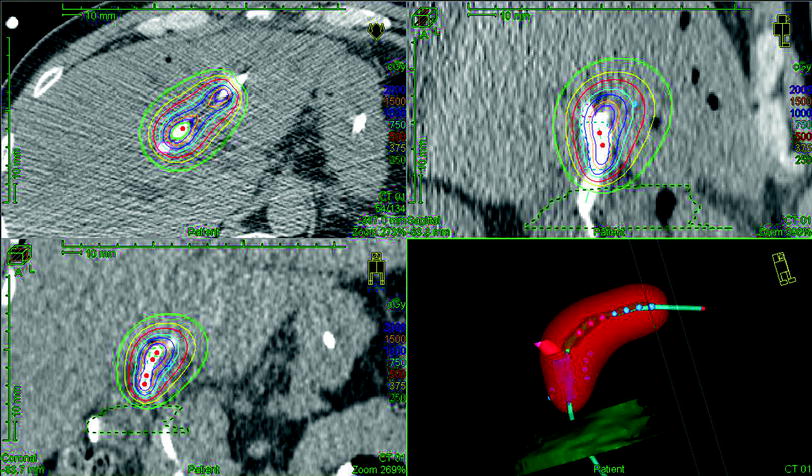
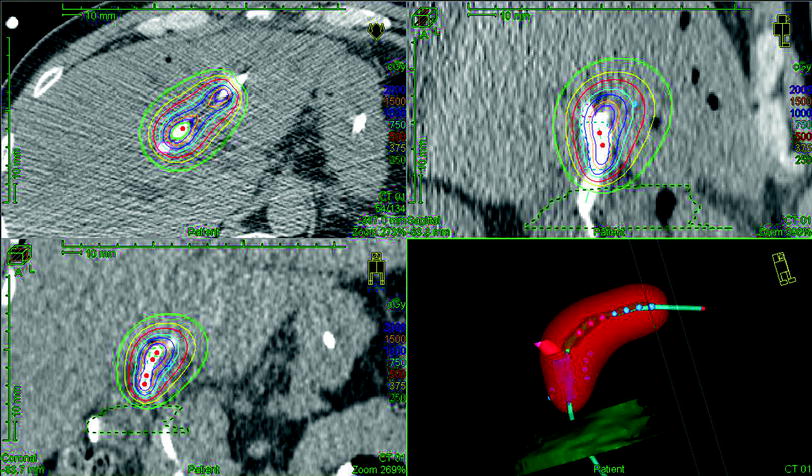
Fig. 2
Planning of intraluminal brachytherapy for biliary malignancy
A transnasal approach using endoscopy at the time of ERCP has also been described [16–27]. This approach allows for internal biliary drainage after sphincterotomy with stent placement and avoids the hepatic puncture associated with the percutaneous technique described above. This approach may be difficult for the wire with the source to traverse the sharp curves in the treatment catheter introduced by this method.
Intraluminal LDR brachytherapy doses (prescribed at 1 cm from the source) are 20–30 Gy as a boost after EBRT or 40–50 Gy as monotherapy given over 1–3 days. HDR doses (again, at 1 cm from the source) are typically 15–20 Gy in 3–4 fractions as a boost or 30–40 Gy in 5–8 fractions, given BID as monotherapy.
Following the procedure, patients should receive broad-spectrum antibiotics to reduce the chance of infectious complications.
2.2 Results
Data supporting the use of intraluminal brachytherapy suggest that this treatment can prolong relief of biliary obstruction and possibly prolong survival.
Early data from Wheeler et al. [11] suggest that patients with malignant biliary obstruction undergoing biliary drainage benefit from intraluminal brachytherapy. In this retrospective report, combining tube drainage with brachytherapy caused a statistically significant “shift to the right” of the survival curve with a median survival of 4 versus 10 months in those treated without and with brachytherapy, respectively [11]. Work by Fletcher, Karani, and Nunnerly have also supported this finding [15, 28, 29].
More recently, Shinohara et al. [30] from the University of Pennsylvania reported a SEER analysis, demonstrating that patients with cholangiocarcinoma undergoing brachytherapy had an improved median survival of 11 versus 4 months. The result was statistically significant and persisted on multivariable analysis. To mitigate any potential bias inherent in an SEER analysis, they also performed a propensity score analysis. When patients were matched by propensity score, the overall survival (OS) benefit of brachytherapy held [30].
A small, randomized prospective trial of 21 patients with inoperable malignant biliary obstruction has been reported in Europe [31]. Patients were randomized to percutaneous insertion of a self-expanding metal stent or the same plus 30 Gy intraluminal brachytherapy delivered over 3 days. The mean stent patency was significantly longer in the brachytherapy group—378 versus 245 days. Additionally, overall survival was noted to be longer in the brachytherapy group—388 versus 298 days (p < 0.05).
When combined with surgical resection, postoperative intraluminal brachytherapy has been reported to increase survival in patients with cholangiocarcinoma [32, 33]. Median survival increased from 8.25 to 19 months with the addition of postoperative brachytherapy. The 1-, 2-, and 3-year OS rates were 36, 18, and 10 % for surgery alone versus 85, 42, and 31 % in patients treated with postoperative brachytherapy (p = 0.0005). Surgical resection with postoperative brachytherapy was also reported to be superior to biliary drainage with intraluminal brachytherapy, with a median survival of 12.3 months.
Montemaggi et al. [19, 20] noted improved locoregional control in patients treated with a combination of concurrent EBRT and intraluminal brachytherapy. Intraluminal brachytherapy combined with EBRT appeared to be superior to EBRT alone and encouraging evidence suggests that intraluminal brachytherapy may favorably impact survival without significant associated toxicity [19]. In contrast, other reports have not supported the use of postoperative intraluminal brachytherapy. A study at Johns Hopkins reported similar survival in patients treated with radiotherapy (a combination of EBRT and intraluminal brachytherapy) and those who did not undergo postoperative treatment with median survival of 18 versus 20 months [34]. Cameron et al. [35] and Kraybill et al. [36] also reported no survival benefit with the use of postoperative brachytherapy.
Data on the role of neoadjuvant intraluminal brachytherapy in regard to liver transplantation in cholangiocarcinoma (CCA) patients is emerging. The LDR intraluminal brachytherapy that was administered at Mayo Clinic in 2005 was the first to utilize an endoscopic insertion of biliary brachytherapy catheters directly through internal biliary stents and without the use of nasobiliary tubes (NBTs) [37]. Survival was reported to be 92 % at 1 year and 82 % at 5 years after liver transplantation for the 38 patients that were treated with neoadjuvant chemoradiation, including biliary intraluminal brachytherapy, followed by orthotopic liver transplantation for cholangiocarcinoma [37, 38]. Furthermore, a complete hepatectomy was found to be curative due to the ability of neoadjuvant chemoradiation therapy (including external beam radiation therapy and intraluminal brachytherapy) to contain and treat the cancer in cholangiocarcinoma patients [37]. Because the patients were treated with multiple therapeutic modalities, it is difficult to establish a definitive causal relationship between intraluminal brachytherapy and improved outcome in patients with cholangiocarcinoma. However, long-term survival for a group of patients with unresectable CCA using neoadjuvant intraluminal brachytherapy alone with chemosensitization reports that 50 % of the patients were alive and disease-free 2.8–14.5 years after liver transplantation [39].
3 Interstitial Brachytherapy
Interstitial brachytherapy involves the placement of radioactive sources or afterloading catheters directly into target tumors or tissues. With hepatobiliary tumors, this is most often done in the intraoperative setting permitting direct visualization of the target. Typically, LDR iodine-125 sources are permanently implanted into the tumor or HDR iridium-192 can be afterloaded temporarily if an appropriately shielded operating suite is available.
3.1 Methods
Small unresectable tumors of the liver can be implanted with iodine-125 (I-125) seeds [40]. A nomograph is used to determine the number and activity of the seeds based on tumor size [41]. After the target area is defined, hollow interstitial needles area placed into the target with 1 cm spacing. The use of intraoperative imaging is encouraged to avoid puncturing large blood vessels and most procedure-related bleeding can be controlled with simple pressure. After all needles are in place, a Mick applicator is used to deposit the I-125 seeds along the path of the needle track with one centimeter spacing between each seed. The Mick applicator allows the needle to be drawn back at predetermined “steps” and individual I-125 seeds deposited at the needle tip. Following seed deposition, the needles are removed. Post-implant CT scans are performed for dosimetric purposes. Doses of 140–160 Gy are typically prescribed with I-125 seeds.
There is also experience with intraoperative HDR [42]. With this technique, the liver is mobilized during laparotomy and several 14-gauge close-ended needles are placed within a non-resectable tumor. Doses in the range of 20 Gy are given in a single fraction, while the patient remains anesthetized and remotely monitored.
For resectable tumors for which margin are a concern (typically adjacent to large vessels), I-125 seeds can be fixed to a two-dimensional substrate and directly opposed to the area at risk [40]. The technique is as follows: I-125 seeds are placed 1 cm apart on a gelfoam sheet trimmed to the dimensions of the tumor bed and then covered with vicryl mesh. The mesh is then sutured directly to the area at risk and, if possible, should be covered by an omental pedicle flap to reduce radiation dose to nearby bowel. This functions as a permanent surface implant delivering a high radiation dose directly to the area at risk.
More recently, CT-guided percutaneous interstitial hepatic brachytherapy has been used [43]. The advantage of this technique is the less invasive nature of the procedure compared with laparotomy.
3.2 Results
Nag et al. [40] reported their results using permanent 125I interstitial implants in a relatively large retrospective study of 64 patients with intrahepatic malignancies that were either unresectable or were incompletely resected. In this study, 58 patients had hepatic metastases from colorectal carcinoma, 4 patients had intrahepatic cholangiocarcinoma, and 2 patients had hepatic metastases from non-colorectal cancers. Plans were designed for a minimum peripheral dose of 160 Gy. The 1-, 3-, and 5-year actuarial control rates for liver disease in these patients were 44, 22, and 22 %, respectively, and the median time to liver recurrence was 9 months (95 % CI, 6–12 months). The overall liver recurrence rate was 75 %, and these were isolated recurrences in 55 % of the patients. Overall, control rates of liver disease correlated with the number of liver metastases: Patients with solitary metastases had a 38 % 5-year control of liver disease; patients with 3 or fewer metastases had a 32 % 5-year control of liver disease; and patients with 4 or more metastases had an 8 % 5-year control of liver disease. Median times to liver recurrence in these subgroups were 17 months (95 % CI, 3–31), 12 months (95 % CI, 2–22), and 6 months (95 % CI, 5–7), respectively. Analysis of the number of implants, implant volume, and MPD failed to show any significant correlation with the control of liver disease. The 1-, 3-, and 5-year survival rates for all patients in this study were 73, 23, and 5 %, respectively, and the median survival time was 20 months (95 % CI, 16–24). The overall 5-year survival rate with no liver metastases was 3 %. Overall survival was found to correlate inversely with the size of the implant volume (p = 0.049); patients with implant volumes of ≤20, 21–64, and ≥65 cc had median survival times of 25 months (95 % CI, 20–30), 14 months (95 % CI, 5–23), and 7 months (95 % CI, 1–13), respectively. Complications in this study were reported in only 6 patients (9 %). There were 2 deaths (3 %) within the 30-day postoperative period; one patient developed a small-bowel fistula distant from the implanted area and died of multiorgan failure, and the second patient died of aspiration pneumonia. Other complications included: a small-bowel obstruction, a small-bowel perforation, a liver abscess, and a wound abscess related to seed implantation.
Thomas et al. [42] from Georgetown University reposted the results of a prospective phase I/II trial. In this study, 22 patients with 24 unresectable hepatic metastasis from colorectal cancer underwent interstitial HDR at the time of laparotomy. Treatment was given to the tumor periphery ranging from 20 to 30 Gy in a single fraction. No acute or chronic toxicity was noted at a median follow-up of 11 months. Actuarial local control was 26 % at 26 months with a median time to local failure of 8 months.
Pech et al. [44] reported a matched-pair analysis of 18 patients with 36 solitary metachronous liver metastasis from colorectal cancer that underwent either percutaneous image-guided HDR interstitial brachytherapy or interstitial laser ablation (ILT). The patients were matched upon tumor size, location, and use of adjuvant chemotherapy after the intervention. No major complications were observed with either technique. At a median follow-up of 14 months, the local control at 1 year was 72 % in the HDR brachytherapy group and 36 % in the patients undergoing ILT (p = 0.004).
A prospective phase II trial that enrolled patients with liver metastases from primary breast cancer was recently reported [45]. Forty-one patients with 115 unresectable tumors were treated with a single fraction of percutaneous image-guided brachytherapy. The median prescription dose ranged from 12 to 25 Gy based upon dose to surrounding critical structures. Treatment planning parameters specified that no more than 33 % of the liver could receive more than 5 Gy. Only one “major” toxicity occurred—a post-procedure hemorrhage requiring a blood transfusion. No decline in liver function tests was noted at a median follow-up of 18 months. Local tumor control was 93.5 %, and overall survival was 60 % at 18 months.
A prospective phase II trial combining hepatic arterial chemotherapy infusion with interstitial HDR brachytherapy was reported by Wieners et al. [46]. Thirty-three patients with unresectable metastases from colorectal cancer were enrolled. A median dose of 15–25 Gy in a single fraction (based on tumor size and surrounding critical structures) was given along with hepatic infusional 5-fluorouracil. At a median follow-up of 28 months, local control was 69 % at 24 months. The median time to progression was 10.5 months. Overall survival was 30 % at 60 months. In terms of toxicity, grade I, II, III, and IV toxicity was noted in 6, 11, 7, and 1 patients, respectively.
A contemporary experience with CT-guided interstitial HDR brachytherapy has been recently reported. Tselis et al. [47] described the results of 36 tumors in 31 unresectable patients with a mix of primary and metastatic hepatic lesions. None of the patients underwent chemotherapy or external radiotherapy to the liver. The dose prescribed was variable but ranged from 30 Gy in 5 fractions, BID to a single fraction of between 7 and 14 Gy. The median prescribed DEQ2 (the total dose expressed as if delivered in standard 2 Gy fractions) was 30 Gy. The local control at 1 and 3 years was 82 and 57 % for all tumors, 79 and 59 % for metastatic tumors, and 88 and 50 % for primary hepatic malignancies. The median overall survival was 14.6 months. Two patients (16.5 %) developed “minor” toxicity (pain, nausea, vomiting) and 2 patients developed “major” toxicity noted to be hemorrhage that required transfusion.
Overall, these results indicate that interstitial brachytherapy is a safe and effective method of obtaining local tumor control for hepatobiliary tumors. These useful techniques are difficult to perform and not widely available. Patients thus need to be referred to specialized centers performing these techniques.
4 Radioembolization (90Y Microspheres)
Radioembolization of liver cancers takes advantage of the unique vascular system of the liver. In normal liver tissue, approximately 70–80 % of the organ’s blood flow is supplied by the portal vein, and the hepatic artery accounts for the rest. This contrasts with both hepatocellular carcinoma (HCC) and metastatic tumors in the liver, in which the hepatic artery supplies approximately 80–100 % of the blood flow [48–50]. This difference in perfusion is exploited by the technique known as radioembolization, whereby radioactive microspheres embedded with a beta-emitting isotope, Yttrium-90 (90Y) are implanted into tumors of the liver by delivering the microspheres through the hepatic artery (Fig. 3). Although first performed in 1960 prior to other forms of embolization not using radiation, [51, 52] commercial agents were not available until the late 1980s [53]. An independent group of international experts from the fields of interventional radiology, radiation oncology, nuclear medicine, medical oncology, and surgical oncology involved with Y90 microsphere therapy, the Radioembolization Brachytherapy Oncology Consortium (REBOC), has recently issued clinical guidelines for Y90 microsphere brachytherapy [54].
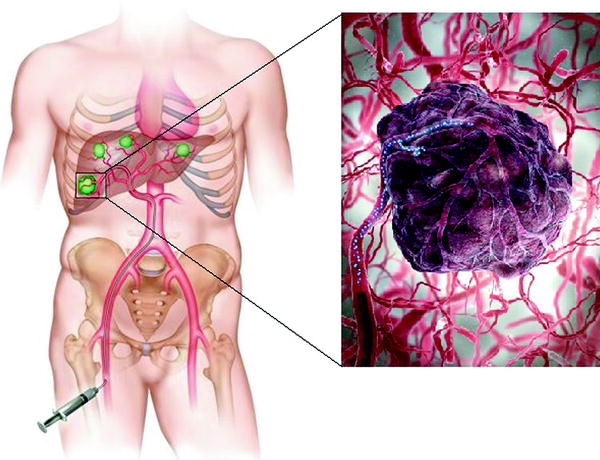
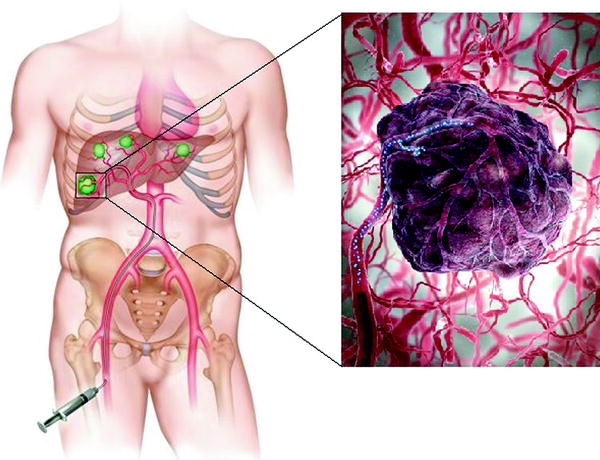
Fig. 3
An injection of microspheres embedded with 90Y into the hepatic artery causes cancer cells to die through the emission of beta particles (Yttrium-90 microsphere radioembolization—cancer treatment. Ria Endovascular. http://www.riaendovascular.com/services-procedures/cancer-interventional-oncology/yttrium-90-radioembolization/. Accessed November 08, 2013)
The use of radioactive isotopes released into blood vessels in the treatment of cancer dates back to the 1940s. In the early years, unsealed sources, primarily 63Zn, 198Au, or radioactive carbon were used [55–57]. However, they could not be localized to a tumor and were, therefore, of limited use due to normal organ toxicity. An early version of resin microspheres containing 90Y also had a problem with leaching of a portion of the radioactive moiety, but this was corrected quickly and is not an issue in modern microspheres. Currently, the use of 90Y microspheres is favored; these are available in two forms: 90Y bound resin microspheres (SIR-Spheres, Sirtex Medical, Australia) and 90Y imbedded glass microspheres (TheraSphere, Nordion, Canada). 90Y is an ideal isotope because it has a short half-life (64.2 h) and is a beta emitter (99.7 %) as it decays to stable Zirconium. Both of the commercially available microspheres contain 90Y, which is produced either by bombarding 89Y in the microspheres with neutrons in a nuclear reactor or using free 90Y to bind irreversibly to resin microspheres. The “hot” radioactive microspheres are delivered to the facility where the treatment is to be performed either on the day of the procedure (resin) or days earlier (glass). Resin microspheres gained premarket approval from the FDA in 2002 for the treatment of hepatic metastases from colorectal adenocarcinoma concurrent with fluorodeoxyuridine (FUDR) hepatic artery infusion. Glass microspheres have use only under humanitarian device exemption (HDE) for the treatment of unresectable HCC. This requires all patients to be enrolled into an IRB-monitored clinical trial. Table 1 outlines the characteristics of each type of microsphere.
Table 1
Properties of resin and glass Y90 microspheres
Parameter | Resin | Glass |
---|---|---|
Trade name | SIR-spheres | TheraSpheres |
Manufacturer | Sirtex Medical, Sydney, Australia | Nordion, Ottawa, Canada |
Diameter | 20–60 micronsa | 20–30 micronsb |
Specific gravity | 1.6 g/dl | 3.6 g/dl |
Activity per particle | 50 Bq | 2,500 Bq |
Number of microspheres average treatment | 15 million | 4 million |
Material | Resin with bound yttrium | Glass with yttrium in matrix |
4.1 Methods
Guidelines regarding the use of 90Y microspheres have recently been published by the REBOC [54] and are summarized here. Because this multidisciplinary technology has been developed by and involves the skill sets of the fields of Radiation Oncology, Interventional Radiology, and Nuclear Medicine, it is strongly recommended that a multidisciplinary team is established that includes individuals with the expertise needed to safely and successfully conduct radioembolization procedures. The team should be able to assume the overall medical management of a cancer patient, perform vascular catheterization, perform and interpret radiologic scans, assume responsibility for the delivery of the 90Y microspheres and be an authorized user, and monitor radiation safety. Typically, institutions have achieved this by combining personnel from various disciplines, including interventional radiology, radiation oncology, nuclear medicine, medical physics, hepatology, surgical oncology, medical oncology, and radiation safety.
Patients should always be evaluated for surgical resection before being considered for 90Y microsphere radioembolization. In addition, the patient’s hepatic disease should represent the bulk of their disease, and they should have a life expectancy of at least 3 months. Relative contraindications include limited hepatic reserve, irreversible hyperbilirubinemia (>2 mg/mL), compromised portal vein (unless selective radioembolization can be performed), and previous radiation to the liver. Goin et al. [58] have published a risk stratification analysis of 90Y glass microspheres from data in 121 patients with unresectable HCC. Retrospectively, the group was divided into low-risk and high-risk groups based on 3-month survival. Seven risk variables were identified as associated with 3-month mortality and were classified as either (1) liver reserve risk factors or (2) non-liver reserve risk factors. The five liver reserve risk factors included bulky disease (tumor volume greater-than-or-equal-to 70 %, or tumors too numerous to count), infiltrative disease (indistinct tumor/liver interface, exhibiting high degree of vascular infiltration on contrast CT), serum transaminase levels greater than five times the normal limit, bilirubin levels greater-than-or-equal-to 2 mg/dL, and tumor volume greater-than-or-equal-to 50 % with serum albumin levels less than 3 g/dL. The two non-liver reserve risk factors included lung dose greater than 30 Gy and a diagnosis of non-HCC disease. Patients grouped into the low-risk group according to this schema had improved survival compared with that of patients at high risk (median survival 466 vs. 108 days).
Prior to treatment with 90Y microspheres, several important studies and procedures should be performed to establish patient eligibility and safety. To evaluate hepatic and renal function, the standard serum laboratory values should be obtained. A three-phase contrast CT and/or a gadolinium-enhanced MRI scan should be performed to evaluate portal vein patency and the hepatic and extrahepatic disease burden. An FDG-PET scan and SPECT imaging may also be useful in measuring hepatic and extrahepatic disease burden and provide information on dosimetry of 90Y delivery (Fig. 4). Arteriograms of the aorta, superior mesenteric, celiac, and right and left hepatic arteries should be performed to evaluate the patient for any anatomic variations in vasculature and to document the perfusion characteristics of the areas of interest. For these studies, percutaneous catheterization is generally preferred over the use of indwelling arterial catheter devices. In order to reduce the risk of unwanted reflux of microspheres into the GI tract, it is also recommended that the gastroduodenal artery and right gastric artery be embolized. As revascularization can occur in a short period of time (days), repeat arteriograms should be performed immediately before the actual administration of 90Y microspheres to make sure that revascularization of prophylactically embolized arteries has not occurred. A 99mTc macro-aggregated albumin (MAA) scan should be performed to evaluate the extent of any extrahepatic shunting; results suggesting radiation exposure to the lungs or gastrointestinal tract greater-than-or-equal-to 30 Gy represent a contraindication to radioembolization with microspheres. When the MAA scan is performed, catheter position and flow rates that are used should be representative of the anticipated catheter position and flow rates of the 90Y infusion. Scintigraphy should be performed within 1 h of MAA administration to prevent false-positive extrahepatic activity due to free technetium. Once the results of these studies are reviewed and approved by the treating team, and there is consensus regarding the planning tumor volume, proposed activity, and optimal catheter placement, treatment with 90Y microspheres may safely proceed.
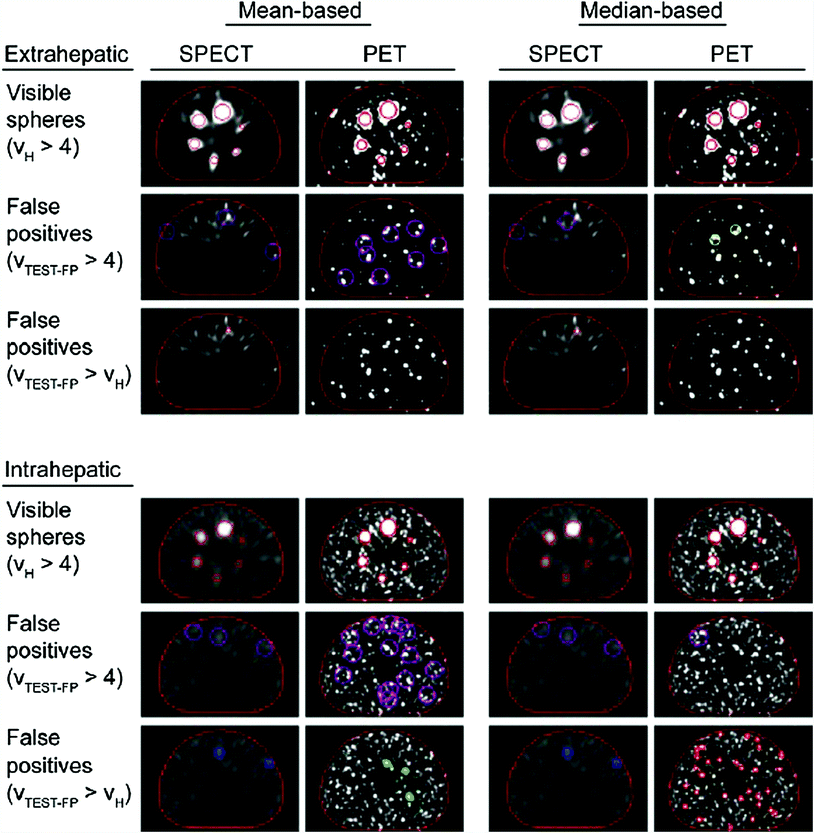
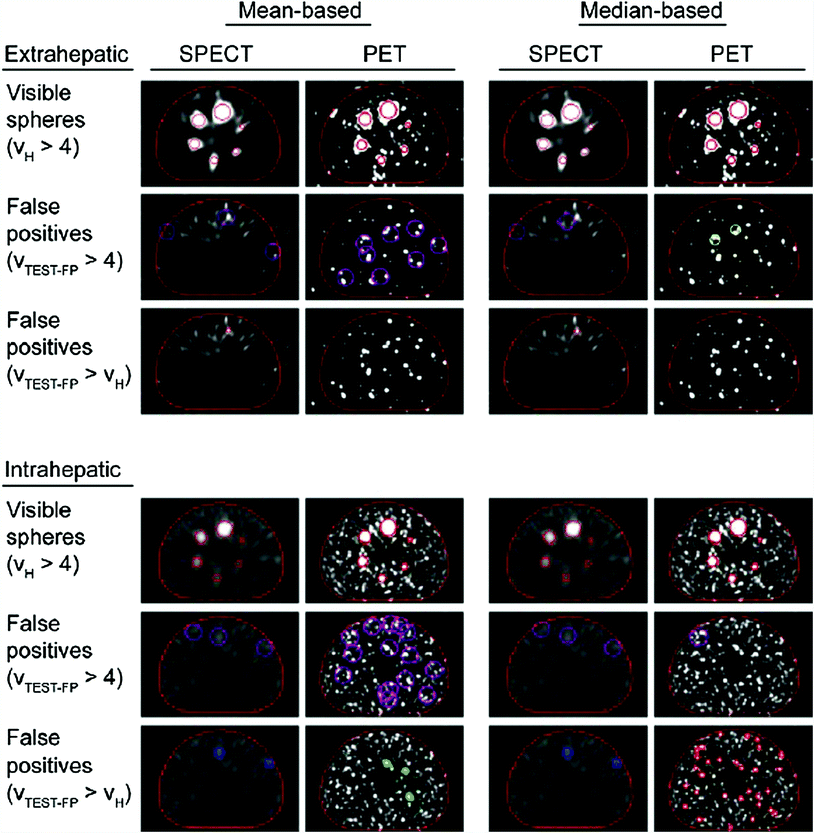
Fig. 4
A visual comparison of FDG-PET versus SPECT imaging for the assessment of intrahepatic and extrahepatic microsphere distribution after radioembolization. FDG-PET imaging often has superior quality than that of SPECT although both methods can provide useful data regarding dosimetry and disease burden [88]
Whole liver or unilobar administrations are both acceptable approaches for 90Y microspheres, and the decision between the two depends on multiple factors. Treating the entire liver in one session is called whole liver delivery, and treating a single lobe is called lobar delivery. Sometimes the entire liver will be treated one lobe at a time, which is referred to as sequential delivery. In sequential treatments, a 30–45-day interval between treatments is generally observed [59–61]. The activity, number of microspheres, and volumes to be treated will vary for an individual patient and differ depending on the type of microsphere being used (Table 1). Resin microspheres are received in bulk, and the individual medical center extracts the desired activity of 90Y calculated for a specific patient from a 3-GBq source vial that arrives on the day prior to treatment. This process differs from that of glass microspheres, which arrive a few days prior to the procedure and all of which (i.e., the entire contents of the vial containing the spheres) are delivered to the tumor.
When choosing an activity, the significant physical differences between the two spheres must be considered: (1) Activity per microsphere: Glass microspheres contain up to 2,500 Bq/sphere and only 1–8 million spheres are delivered for the typical patient. This number of glass spheres is not sufficient to cause significant embolization in the main hepatic arteries. Resin microspheres contain approximately 50 Bq/sphere; thus, an average treatment contains 15–25 million spheres, a number that can cause embolic effects in the arteries. (2) Embolic effect on dose delivery
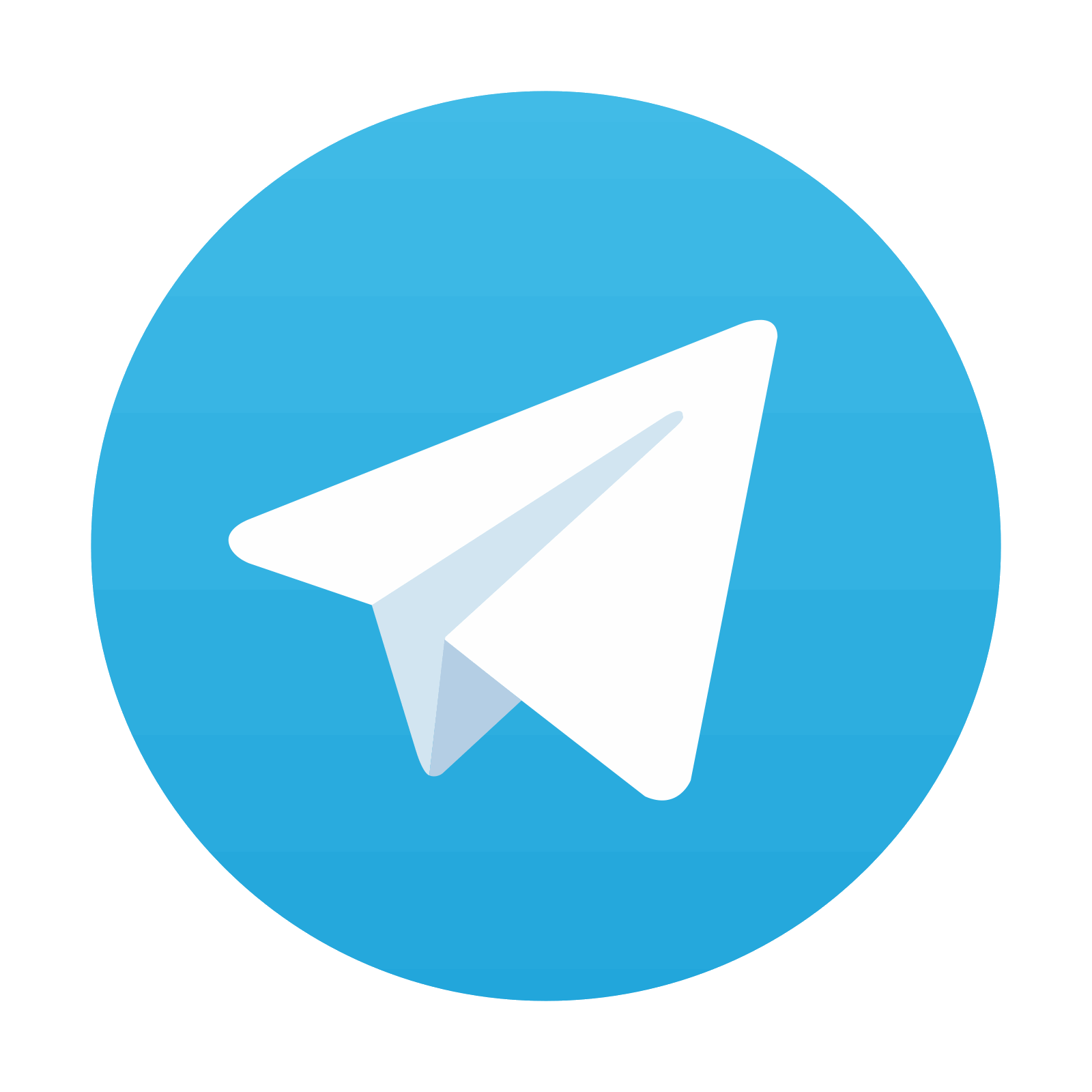
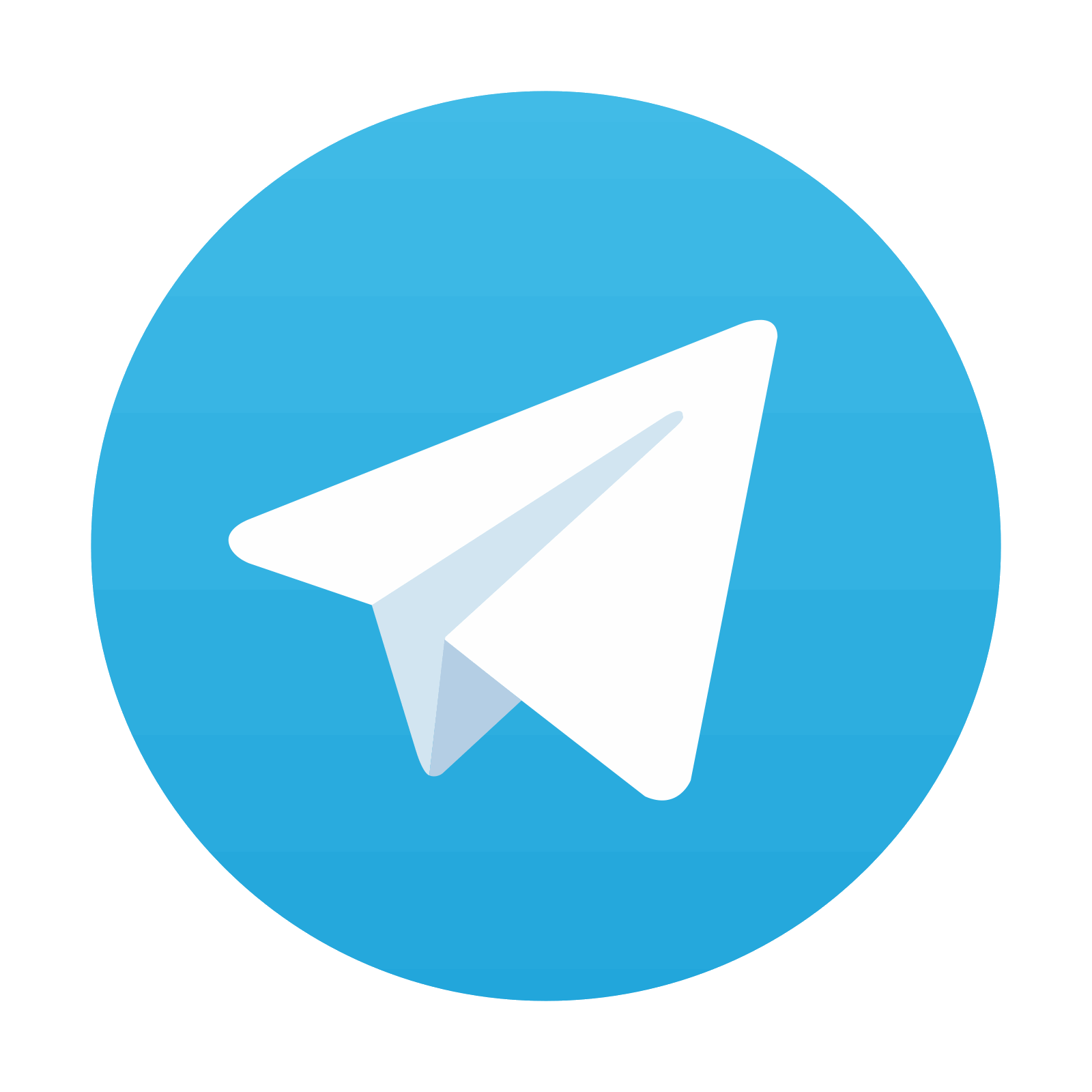
Stay updated, free articles. Join our Telegram channel
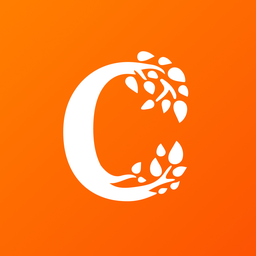
Full access? Get Clinical Tree
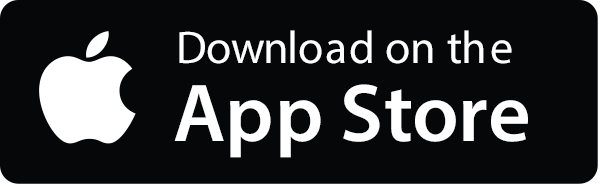
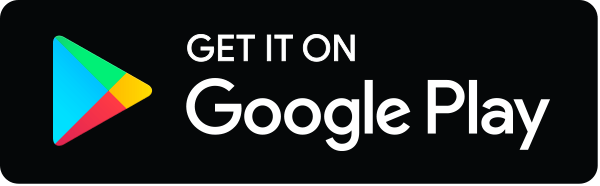