IVIM fMRI: Brain Activation with a High Spatial Specificity and Resolution
More than three decades ago, intravoxel incoherent motion (IVIM) contrast was proposed as a potential mechanism sensitive to blood flow changes during brain activation. While not as widely used as the blood-oxygenation-level-dependent (BOLD) contrast, which arises from venous networks, IVIM may offer improved spatial localization to smaller vasculature (e.g., arterioles and capillaries) more closely tied to the sites of neural activity. However, inherent high spatial specificity can only be realized and demonstrated if the IVIM images can be acquired at high spatial resolutions. To date most prior work on IVIM fMRI relied on diffusion-weighted single-shot EPI techniques, which are constrained to low spatial resolutions. Recent advances in multishot diffusion MRI, such as that using multiplexed sensitivity encoding (MUSE), have built a solid technical foundation to enable IVIM fMRI at much higher spatial resolutions. This new advancement can be incorporated with previous theoretical framework and acquisition strategies on IVIM to investigate functional motor activations at both high spatial resolutions and high spatial specificity in the finger homunculus. New results along this front are also illustrated in this chapter. It is hoped that such progress will allow IVIM-based functional brain activation in the cortical gray matter to be investigated in the finest-grained detail, further complementing the current fMRI practice based on the BOLD contrast.
5.1 Introduction
The contrast mechanism based on the IVIM [1] has seen extensive growth over the past three decades, reaching far beyond the original realm of diffusion magnetic resonance imagery (MRI). Within the realm of functional MRI (fMRI) [2–7], IVIM contrast has provided unique contributions thanks to its high sensitivity to moving proton pools, for example, blood flow changes, upon brain activation.
Over the past 20 some years, BOLD contrast [4] has been extensively used in fMRI. However, its spatial specificity can be influenced mostly by large veins [8] where the oxygenation level changes are the greatest, which are often spatially distant from the sitesof neural activity. Usinginvasive opticalimagingintraoperatively in humans in vivo, Hochman and Haglund [9] have shown that blood-oxygenation-level changes primarily occur in large veins, while blood volume changes are more focal in small vessels and spatially colocalized with neuronal activities (Fig 5.1).
Figure 5.1 Derived from optical imaging in the human sensory cortex during sensory stimulation, contrast in blood volume is spatially colocalized to upstream small vessels and neuronal activities, while blood-oxygenation-level changes are predominantly located in the venous network.
Within the fMRI community, parallel development in cerebral blood flow (CBF) and cerebral blood volume (CBV) contrasts has been developed and applied over the past two decades. Specifically, by employing an arterial spin labeling (ASL) scheme [10–12], perfusion-weighted images can be made sensitive to the CBF changes from upstream arterial networks to the capillaries. Because of the exchange mechanism in the capillaries and the diminishing sensitivity in large veins due to T1 recovery, optimized perfusion contrast is believed to have a better spatial localization ability [12, 13] compared to BOLD imaging methods. This finding was also confirmed in animal models, which demonstrated the existence of the orientation columns in the visual cortex of cats [14]. However, perfusion imaging does have limitations, most notably the necessity for the wait time for the labeled upstream blood to arrive at the imaging plane, the often-undetermined mean transit time, and the inevitable T1 recovery, all of which lead to a low temporal resolution and limited spatial coverage. Researchers have also developed CBV contrast to image brain activation [15, 16], which are believed to be originated from small-vessel networks (e.g., arterioles). While these techniques have been shown to provide improved spatial colocalization with neuronal activities, they may be limited by temporal throughput (due to the use of inversion pulse) or require contrast agents.
Similar to CBF and CBV contrasts, the IVIM mechanism can be made sensitive to functional changes in the vasculature. The apparent diffusion coefficients (ADCs) derived at low b values include perfusion-driven IVIM effects. This differs from the ADC derived at very high b values [17, 18] where direct neuronal activation related to potential cell swelling was probed. At low b values, a simple signal classification based on the static ADC can be used to differentiate highly mobile proton pools (e.g., those in large vessels and cerebrospinal fluid [CSF]) from those that are less mobile (e.g., those in brain tissue and small vessels), as illustrated in Fig 5.2. Thus, if the ADC increases upon brain activation due to higher blood flow and volume, it is possible to apply dynamic ADC imaging to capture this increase.
Figure 5.2 A simple signal classification based on static ADC values generally clustered in large vessels, CSF, small vessels, and brain tissues.
A number of groups, including ours, reported that dynamic ADC imaging at low b values can be used for image changes in blood flow upon brain activation [19–22]. Such dynamic use of the moderate diffusion weighting strategies is sensitive to the IVIM and differs from early studies that simply employed static weighting strategies to eliminate proton pools of large mobility [23, 24]. In addition, it is not sensitive to the extravascular component of large vessels as the parenchyma tissue near large vessels does not change mobility over time. Furthermore, it is possible that, by setting an appropriate b factor range for IVIM weighting, ADC contrast can be made uniquely sensitive to the capillary networks where the increased flow within the lumen and exchange with the interstitial space give rise to significant ADC changes. Compared to the traditional BOLD methods, dynamic ADC contrast is advantageous in that (i) it does not require an ultrahigh field, as it is detecting the blood mobility changes, (ii) it has a higher contrast-to-noise ratio (CNR) and lower power consumption compared to the spin-echo BOLD method, which is believed to be another alternative for capillary localization, and (iii) it has the potential to be sensitive to small-vessel networks if the appropriate b factor range is used. Dynamic ADC contrast is also advantageous compared to another popular fMRI method using CBF contrast in that (i) it does not require the long transit and exchange time between tagging and image acquisition and thus could afford much higher temporal throughput and (ii) it intrinsically has a much larger spatial coverage.
As shown in previous reports (e.g., Ref. [22]), appropriate ranges of low b values can generate IVIM contrast highly sensitive to brain activation. Specifically, moderate diffusion weighting (i.e., with b factors ranging from 0 to ~200 s/mm2) has been shown to be primarily sensitive to the IVIM changes in the vasculature. Furthermore, IVIM activations can be classified on the basis of their ADC values to infer the origins of their large or small vessels (Fig 5.3).
Figure 5.3 Concurrent BOLD and ADC activation maps, with ADC values classified in various ranges, from small to large vessels.
Further evidence of the IVIM origin of ADC contrast has been obtained through temporal analysis of ADC activation. Studies [21, 25] have shown that peak ADC activation temporally precedes peak BOLD activation by approximately 2 s (shown in Fig 5.4), with both overlapping and spatially distinct activated regions, suggesting that it mainly originates from capillaries and upstream arterial networks, as opposed to BOLD contrast, which originates from capillaries and downstream venous networks. By selecting an appropriate baseline b factor (e.g., 20 s/mm2), the signal contributions from large arteries with ahigh blood velocitycan be eliminated. By acquiring two or more b factors across an appropriate range, the ADC contrast can then be tuned to increase the sensitivity toward small-vessel networks (i.e., arterioles and capillaries). These networks are more closely tied to the sites of neural activity and can thus achieve improved functional localization [25, 26].
Figure 5.4 Temporal histogram of ADC and BOLD activation across all pixels. The peak ADC activation precedes peak BOLD activation by approximately 2 s, confirming its upstream origin toward the arterial side.
To this end, it was concluded that the IVIM contrast mechanism can complement the existing BOLD contrast in fMRI studies, thereby improving the colocalization with the underlying neuronal activities and enabling the investigation of small-vessel networks.
5.2 IVIM fMRI Needs a High Spatial Resolution
While the IVIM contrast mechanism has the advantage of improved spatial localization in small-vessel networks, earlier applications relied on the traditional single-shot diffusion-weighted echo-planar imaging (EPI) technique to acquire the time-course images while preventing severe motion-induced artifacts. Due to transverse relaxation, the data readout window in EPI acquisitions is limited in duration, making it difficult to achieve the high spatial resolution needed to realize the full benefit in spatial specificity for IVIM fMRI using single-shot EPI. Parallel imaging at a high acceleration factor can potentially reach a very high spatial resolution, but at the cost of a reduced SNR. Ideally, if multiple excitations (shots) can be used, it would remove the constraints on the spatial resolution and SNR. However, due to a heightened motion sensitivity inherent in diffusion weighted acquisitions, shot-to-shot phase variations would result in motion-induced artifacts that render the final images unusable.
Over the last decade, remarkable progress has been made in achieving high-resolution diffusion MRI using multiple excitations, such as multishot EPI. Techniques using a segmented readout window or navigator echoes [27, 28] have been highly effective in reducing the phase variation between shots, but with the potential cost of increased imaging time. A new class of multishot diffusion MRI, termed multiplexed sensitivity encoding (MUSE) [29], takes advantage of the now-common large phased-array coils to generate inherent phase maps to account for intershot motion artifacts in an efficient manner. A simple example using a two-shot diffusion- weighted EPI acquisition, shown in Fig 5.5, graphically illustrates the effectiveness of phase correction using the MUSE technique. Specifically, the advent of receive coil arrays allows researchers to construct images with partially sampled data through sensitivity encoding (SENSE) [30]. As a result, the k-space data in each individual excitation from a multishot acquisition is identical to a SENSE-accelerated scan; thus, it can be reconstructed into a complete image using SENSE in a straightforward manner, shown in the left column of Fig 5.5 for each segment of the two-shot EPI acquisition. Subsequently, the maps of phase discrepancy can be determined on the basis of these two individually reconstructed images (blue box, middle column). This map of phase discrepancy can then be fed back into the original data to improve the conditioning of the matrix inversion to generate the final high-quality image (green box, right column). This final image offers a drastic improvement in image quality as compared to the original image without corrections (red box, middle column).
Figure 5.5 Illustration of MUSE reconstruction using a two-shot diffusion- weighted EPI as an example.
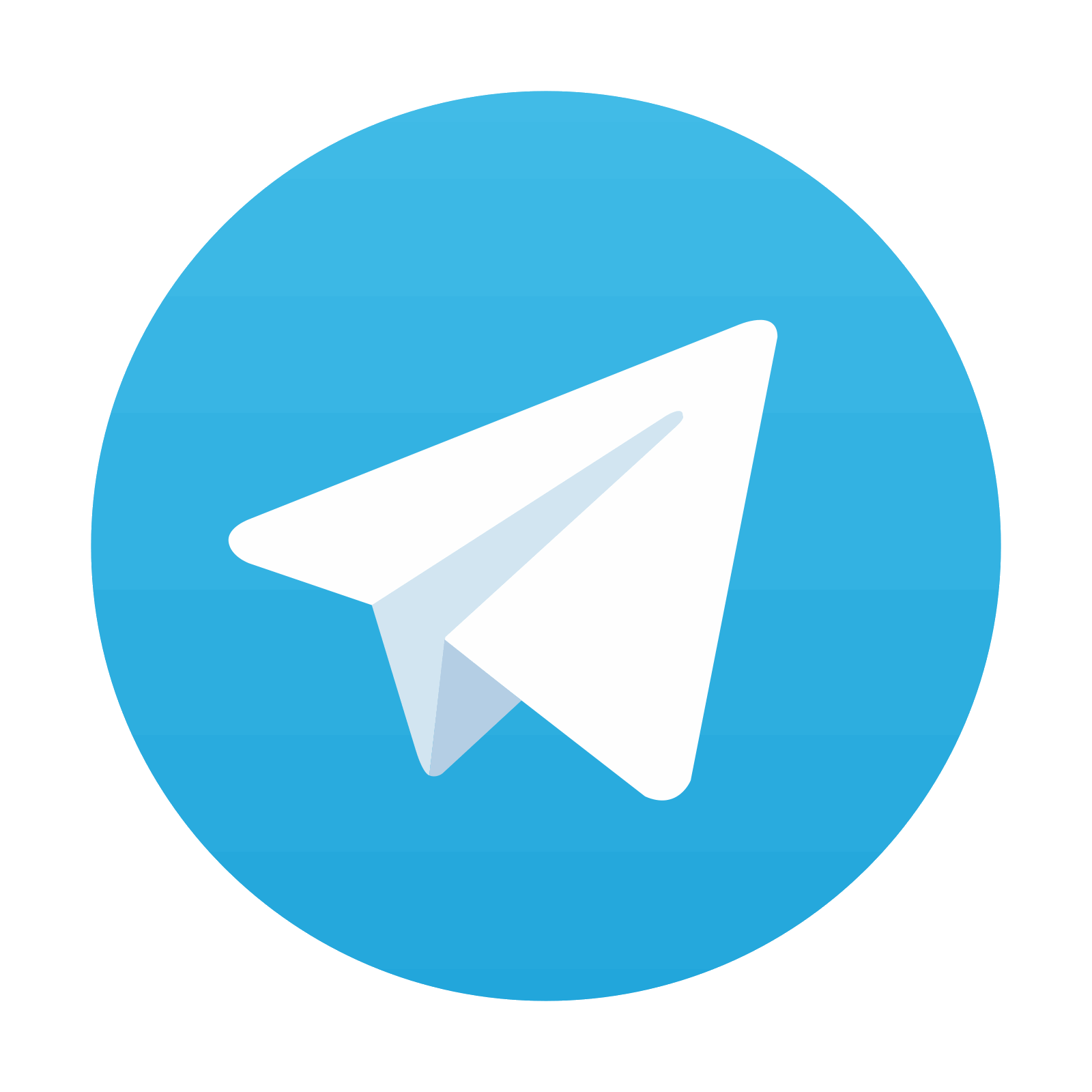
Stay updated, free articles. Join our Telegram channel
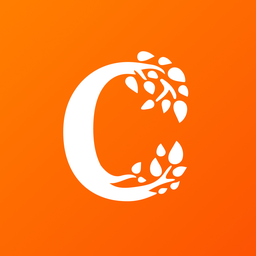
Full access? Get Clinical Tree
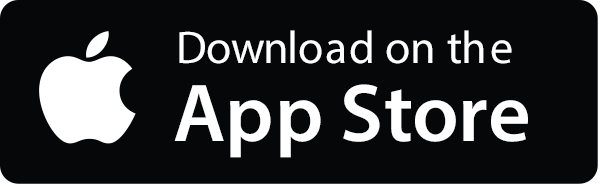
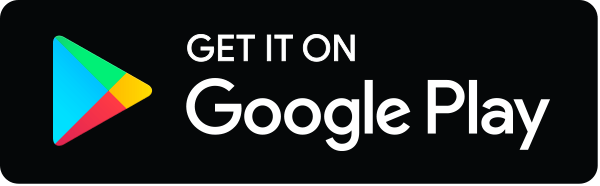