IVIM MRI in the Kidney
13.1 Introduction
The kidneys are the best perfused organs of the human body. They receive 30% of the circulating blood volume, while weighing <1% of the total body mass. The renal main function is filtration of blood and elimination of substances to maintain hemostasis of the body. Anatomically, the renal parenchyma consists of two major structures: the peripheral, relatively thin renal cortex directly beneath the renal capsule and the inner renal medulla. The functional unit of the kidney is the so-called nephron (spanning both the cortex and the medulla), consisting of a glomerulus with its proximal tubule, loop of Henle, distal tubule, and collecting duct. Glomerular filtration takes place in the cortex, which receives approximately 90% of the renal blood flow. While approximately 20% of the blood volume is extracted (extraction fraction), only a small fraction is ultimately excreted. Resorptive tubular processes take place in the medulla, which is supplied by efferent vessels. Due to its relative hypoperfusion, the medulla works almost under hypoxic conditions and thus oxygen saturation is susceptible to changes in perfusion. Perfusion and filtration are autoregulated and not necessarily proportional.
The glomerular filtration rate (GFR in mL/min./body surface) is regarded as the quantitative metric for kidney function. However, insulin clearance, which is regarded as the ideal quantification method, requires serial blood drawings and is thus not routinely performed. Estimation of GFR with the Cockraft–Gault or the modification-of-diet-in-renal-disease (MDRD) formula is relatively precise in the case of reduced kidney function but not when it is in the normal range. Furthermore, they do not allow for the assessment of single side renal function or changes in perfusion and ultrastructure.
Noninvasive magnetic resonance imaging (MRI) methods, such as diffusion-weighted imaging (DWI), have been proposed to assess renal function and tissue integrity. The apparent diffusion coefficient (ADC) is typically reduced in the case of acute and chronic renal disease, such as acute ischemia-reperfusion, pyelonephritis, or diabetic nephropathy [1].
Intravoxel incoherent motion (IVIM) MRI appears particularly promising for the investigation of renal pathologies as it allows the differentiation between perfusion and tissue integrity on the basis of pseudodiffusion and tissue diffusion, respectively. The pseudodiffusion effect is expected to be particularly strong and relatively easy to probe, as the kidneys are extremely well perfused. Furthermore, assessment of tissue integrity is of clinical concern, as invasive biopsy, with its complications, such as hemorrhage and infection, is the only method to gain insight into renal ultrastructure. IVIM metrics may thus be beneficial in the case of renal transplant rejection as well as acute and chronic kidney disease. However, several challenges have to be overcome. First, the kidneys are the organs that undergo the most geometric changes during respiratory motion, including in- and through-plane motion as well as rotation and deformation [2]. Secondly, diffusion as a three-dimensional process is strongly anisotropic in the renal medulla, so diffusion measurements in a single direction may strongly vary depending on the gradient direction [2].
The most important challenge, as in other organs, is to maximize reproducibility and variability of the IVIM experiments to obtain reliable and robust results, which can be interpreted in a transversal and longitudinal fashion.
13.2 Data Acquisition and Processing
As explained above, IVIM MRI of the kidneys is worthwhile yet challenging. In this section we will describe the most important aspects regarding the design of IVIM sequence protocols for applications in the kidneys and the processing of the acquired data.
13.2.1 Pulse Sequence and Acquisition Parameters
Most often, renal diffusion-weighted MRI (DWMRI) is performed using twice-refocused spin-echo sequences with bipolar diffusion gradient lobes and fat suppression, that is, with the pulse sequence [FatSat] – 90° – G + – 180° – G – – G + – 180° – G – – [EPI readout]. The bipolar gradients reduce image artifacts caused by eddy currents [3] at the expense of a slight loss in the signal-to-noise ratio (SNR) compared to the conventional, once-refocused Stejskal–Tanner [4] sequence. The echo planar imaging (EPI) data readout is utilized in renal IVIM MRI for its speed and robustness against motion artifacts and is generally employed in the single-shot mode. Multishot EPI readouts have been shown to improve image quality in the kidneys (higher spatial resolution and less geometric distortion) [5–7] by shortening the echo train length (ETL), however, they require motion compensation techniques and result in substantially prolonged scan times. Further methods routinely used in renal DWMRI to shorten the ETL and thus reduce magnetic susceptibility artifacts include partial-Fourier [8] and parallel imaging techniques, such as generalized autocalibrating partially parallel acquisition (GRAPPA) [9] and sensitivity encoding (SENSE) [10]. As an alternative to EPI acquisitions, single-shot fast spin-echo (turbo spin-echo) pulse sequences have been proposed for renal IVIM acquisitions [11, 12], resulting in reduced distortion artifacts but increased blurring in comparison to EPI.
The field of view (FOV) is usually extended to the patient’s abdominal circumference to avoid folding artifacts. For both coronal and axial image orientations this results in in-slice dimensions between 320 and 400 mm. A coronal orientation allows for a faster full kidney coverage using fewer image slices, thereby saving scan time. However, with the advent of simultaneous multislice MRI, this advantage might be nullified in the future [13]. The resolution chosen should be high enough (approximately 2–3 mm in-slice) to allow for a delineation of the renal cortex and medulla, while yielding adequate SNR to facilitate robust IVIM parameter estimates. The slice thickness may be increased for an improved SNR and faster full kidney coverage; however, an excessive thickness (>6 mm) can lead to undesirable partial-volume effects and image blurring.
As with all IVIM applications, the informative value of the determined parameters depends on the b value set used for imaging. We recommend performing renal IVIM measurements using at least eight b values, with no less than five b values between 0 and 200 s/ mm². Since the tissue diffusivity D is relatively high in the kidney (see Table 13.1), the largest b value should not exceed 800 s/mm² to avoid unwanted noise floor effects [14] and to keep echo times (TEs) short; at the same time, the largest b value should not be substantially lower than 700 s/mm² to enable a precise and accurate estimation of the water diffusion coefficient, D. The kidneys, especially in the medulla, have also been shown to exhibit a markedly anisotropic behavior in DWMRI [2, 15]. Renal IVIM measurement should therefore always be performed using three (or more) orthogonal diffusion gradient directions to base the parameter estimation on trace images. Typical acquisition times for IVIM MRI protocols range from 5 to 12 min.
Table 13.1 Reported IVIM parameters in healthy subjectsa
a (N) Number of healthy subjects; (b values) Number of b values and range (min..max); (–) No data available
b Biexponential model
c Combined values from left and right kidneys
d Only Levenberg–Marquardt (LM) algorithm (from supplemental material)
e Combined values from triggered and nontriggered acquisitions with image registration
f Combined values from free-breathing and navigator-controlled acquisitions
g Combined values from “predonation” and “precept” kidneys (from supplemental material)
h ”Baseline” values
13.2.2 Respiratory Motion
Respiratory motion is abundant in the kidneys, making a suitable compensation strategy key to generating meaningful results. The consequences are most obvious in the coronal orientation, since respiratory motion occurs mainly in the head-foot direction.
Figure 13.1 shows ADC maps calculated from a motion-corrected (left) and an uncorrected (right) free-breathing DW image series.
Figure 13.1 Effect of respiratory motion on ADC parameter maps. Voxel-wise ADC calculation using the uncorrected image series (right) leads to pronounced artifacts at the kidney periphery (arrow). Correcting the image series by two-dimensional rigid registration removes the artifact and improves image sharpness.
As the uncorrected kidney moves up and down throughout the image series due to respiration, the voxel-wise signal behavior becomes erratic at the kidney periphery, resulting in artifacts in the calculated ADC maps.
In the presented example, motion was corrected using a two- dimensional (in-plane) image registration algorithm after data acquisition. Image registration may be performed using rigid or affine transformations based on a predefined similarity measure. If multiple diffusion gradient directions and averages have been acquired, images should be registered before averaging and calculation of the trace to improve image sharpness and general data quality. The respiratory motions of the left and right kidneys may not be synchronous, especially if one of the kidneys is transplanted or treated surgically. Therefore, image registration should be performed for each kidney individually.
The effects of respiratory motion can also be diminished during data acquisition by performing breath-hold imaging or triggering of the pulse sequence. However, breath-hold imaging is of limited use in IVIM measurements since it requires patient cooperation and offers only brief intervals for scanning during each breath-hold. For a robust IVIM parameter estimation in the kidneys, it is essential to acquire images at multiple b values (>8 preferably), which results in total acquisition times of several minutes, making multiple breath- hold cycles necessary. The resulting scan times offer a negligible advantage over triggered imaging, which provides greater patient comfort. Respiratory triggering uses external devices (abdominal pad, belt transducer, etc.) or the MR signal itself (navigator) to infer the state of the breathing cycle and executes the pulse sequence accordingly. The total scan time is thereby dependent on the breathing pattern and may vary between patients. Similar IVIM results have been reported in a direct comparison of free-breathing and navigator-controlled abdominal acquisitions [16]. Regardless of the chosen compensation strategy, image registration after data acquisition is strongly recommended to remove residual motion.
13.2.3 Data Analysis
IVIM parameters in the kidneys are most often estimated on a voxel- wise basis and subsequently reported as mean or median in manually defined regions of interest (ROIs). The ROIs should be defined to encompass either cortex or medulla solely, as they exhibit different signal behaviors in DWMRI measurements and the determined diffusion parameters may be of a different diagnostic value. Vascular structures and collecting systems should be excluded from analysis, and renal masses are to be investigated separately. To reduce the IVIM parameter variability due to noise, segmented biexponential fitting approaches [17–19] are commonly used for IVIM MRI in the kidneys with a cutoff b value of 200 s/mm².
13.3 Healthy Kidneys/Peculiarities
To apply and evaluate IVIM measurements related to different pathological changes of the kidneys, the ranges and mean values of the IVIM parameters (tissue [water] diffusion D, pseudodiffusion D*, and perfusion fraction f) in healthy kidneys of normal subjects must be known. Over the last years, these values have been determined in a relatively large number of renal IVIM studies in healthy subjects. A selection of 13 studies is presented in Table 13.1; included in this overview were studies using at least 10 healthy subjects, data evaluation in the renal cortex or the medulla, and relatively typical IVIM acquisition parameters (e.g., at least eight different b values and a maximum b value between 700 and 1000 s/mm²). Of these 13 studies, 5 were performed at 1.5 T and 8 at 3 T. Most studies applied diffusion weightings in three orthogonal directions, the TEs of the IVIM acquisition varied between 52 and 93 ms, and acquisitions were performed in breath-hold, during free breathing, or with respiratory triggering. Image orientation was coronal in nine studies, transversal in four studies, and sagittal in one study. All studies determined the tissue diffusion coefficient D, and the perfusion fraction f, at least in the renal cortex; the majority of these studies provided also results for the pseudodiffusion coefficient D*. The total number of subjects in all included studies is 202.
Compared over all included studies, the evaluated IVIM parameters show a certain variation that is lowest for the tissue diffusion coefficient D and highest for the pseudodiffusion D*. The weighted mean values of D over all studies are (1.89 ± 0.13) ¥ 10–3 mm2/s in the cortex and (1.78 ± 0.17) ¥ 10–3 mm2/s in the medulla. In all included studies, D is higher in the cortex than the medulla (in one case, equal values are reported). However, the ranges of the reported mean values over all studies (bottom row of Table 13.1) are quite large, with a substantial overlap of the results in the cortex and the medulla.
The weighted mean values of the perfusion fraction f over all studies are 20.7% ± 7.9% in the cortex and 18.6% ± 7.2% in the medulla. In all but one study, the mean f is reported to be greater in the cortex than in the medulla.
The pseudodiffusion coefficient D* shows by far the greatest variability over all studies, with study mean values ranging from 12 to 84 ¥ 10–3 mm2/s. The weighted mean values over all studies are (28 ± 14) ¥ 10–3 mm2/s in the cortex and (27 ± 21) ¥ 10–3 mm2/s in the medulla. There is no clear tendency whether D* is greater in the cortex than in the medulla.
One potential reason for these large variations in D* is the influence of the postprocessing strategy and fitting algorithm. This is illustrated, for example, in the study by Barbieri et al. [19] where averaged D* values between about 15 and >200 ¥ 10–3 mm2/s are calculated for the same data but with different biexponential fitting algorithms. Another reason for these variations may be the existence of a third, very fast diffusion component (>200 ¥ 10–3 mm2/s), as shown by Wurnig et al. [30]; depending on the acquisition parameters (in particular, the number of b values and the distribution of very low b values, <50 s/mm²), this third component may influence the fitting results to varying degrees.
Several physiological properties are also supposed to influence the IVIM resultsinhealthysubjects, such as the renalhydration status, the respiration, or the pulsatile arterial blood flow. Sigmund et al. demonstrated that the diffusion coefficients D and D* increase after hydration or a furosemide challenge [27], whereas other groups did not find significant differences in conventional diffusion parameters at different hydration states [31, 32]. To assess the relevance of respiratory motion for IVIM measurements, Jerome et al. compared free-breathing and respiratory-triggered IVIM acquisitions without finding significant differences of f, D, or D* [16]. The influence of the varying blood flow during the cardiac cycle on IVIM parameters has been analyzed by Wittsack et al. [33]; they show that in particular the perfusion fraction f (in addition to the pseudodiffusion D*) is substantially increased if the IVIM acquisition is electrocardiogram (ECG)triggered at the time of the maximum pulsatile flow.
A recent development in renal IVIM measurements is the analysis of the anisotropy of the pseudodiffusion D*, that is, a pseudodiffusion tensor imaging approach. This was first proposed by Notohamiprodjo et al., who demonstrated a substantially greater medullary D* in axial than in radial direction, that is, a significant pseudodiffusion fractional anisotropy FA(D*) = 0.42 ± 0.02 in the medulla as opposed to a more anisotropic perfusion behavior in the cortex with FA(D*) = 0.09 ± 0.04 [15]. The remaining IVIM metrics D and f display a directional dependence as well (Fig 13.2). Similar results were described by Liu et al. [20], Ye et al. [34], and Hilbert et al. [35].
Figure 13.2 Polar plot analysis of D (a, b) and f (c, d) in the medulla and the cortex, respectively, in a healthy volunteer. The red dots indicate the angular distribution of the mean averaged over multiple regions of interest. The distribution shape indicates a strong anisotropy in the medulla and little anisotropy in the cortex [15].
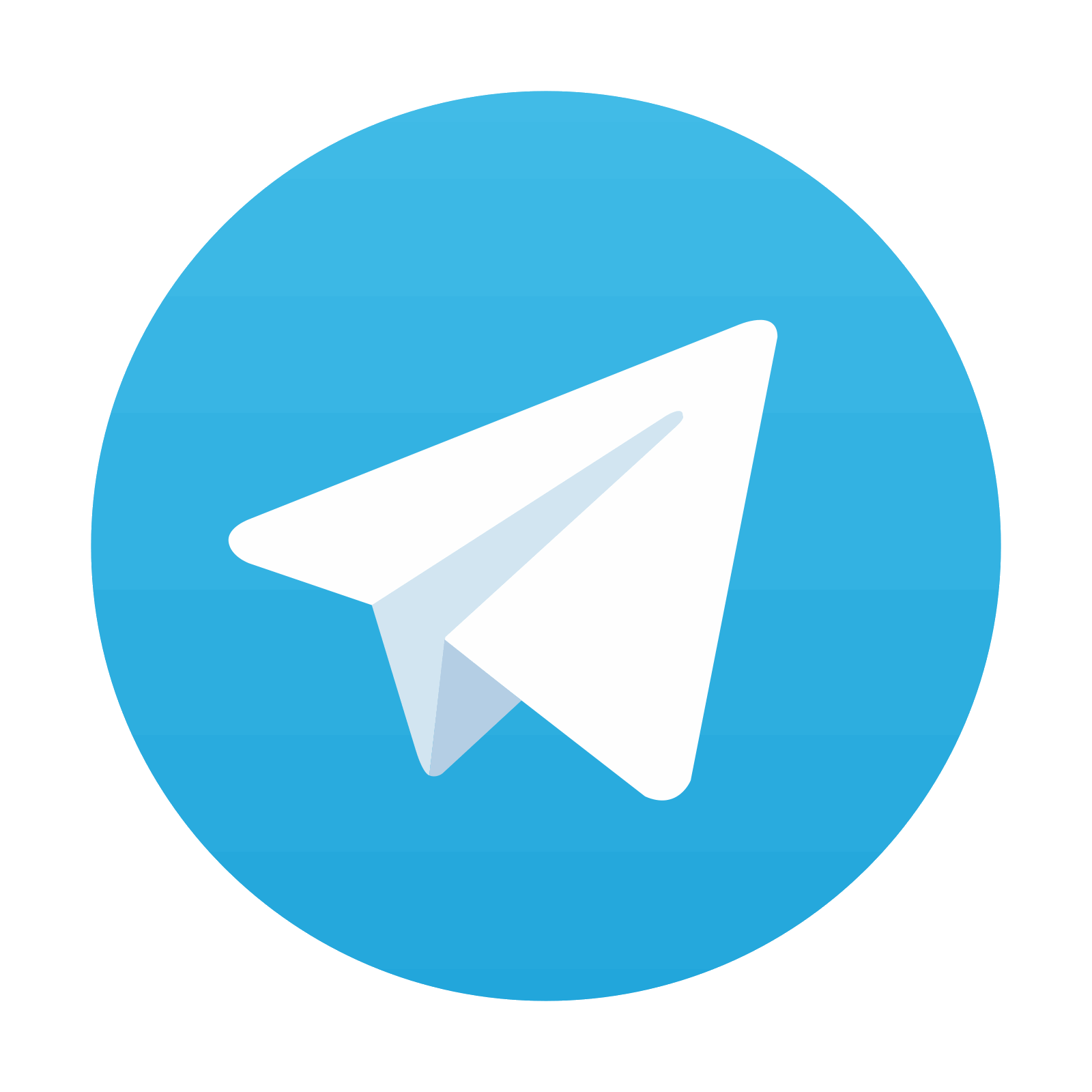
Stay updated, free articles. Join our Telegram channel
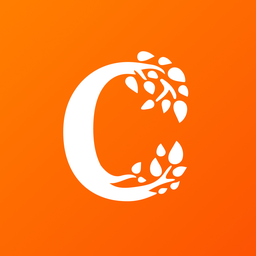
Full access? Get Clinical Tree
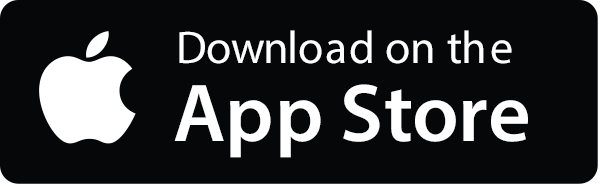
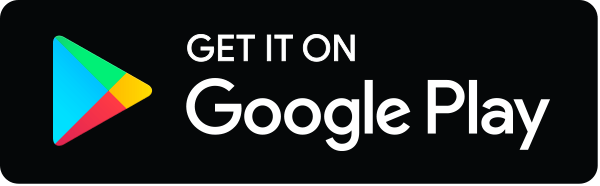