shows the cause-specific cumulative late mortality. Risk of death remains highest for recurrence of original disease, but 14% of deaths from this cohort are now from subsequent malignancies (4).
populations. Nondividing cells exist in several compartments: for example, some may be found resting in G0 phase, although not all of these are fully quiescent since some populations appear to be capable of re-entering cell cycle if the tissue is challenged or stimulated (6). Clinically, it is clear that the greater potential for growth in pediatric tissues will mirror many of the adverse effects of radiation therapy since insult will absolutely compromise growth potential and manifest as impaired development. In fact, the injury that can occur in a pediatric tissue may not become manifest until that tissue rapidly develops. An example of this is the impairment of bone growth seen during adolescence but due to radiation many years before.
Table 19.1 Tolerance Dosages, TD5-TD50 (Fractionated Dosage, Whole or Partial Organ) | ||||||||||||||||||||||||||||||||||||||||||||||||||||||||||||||||||||||||||||||||||||||||||||||||||||||||||||
---|---|---|---|---|---|---|---|---|---|---|---|---|---|---|---|---|---|---|---|---|---|---|---|---|---|---|---|---|---|---|---|---|---|---|---|---|---|---|---|---|---|---|---|---|---|---|---|---|---|---|---|---|---|---|---|---|---|---|---|---|---|---|---|---|---|---|---|---|---|---|---|---|---|---|---|---|---|---|---|---|---|---|---|---|---|---|---|---|---|---|---|---|---|---|---|---|---|---|---|---|---|---|---|---|---|---|---|---|
|
Table 19.2 Common Chemotherapy Late Effects | |||||||||||||||||||||||||||||||||||||||||||||||||||||||||
---|---|---|---|---|---|---|---|---|---|---|---|---|---|---|---|---|---|---|---|---|---|---|---|---|---|---|---|---|---|---|---|---|---|---|---|---|---|---|---|---|---|---|---|---|---|---|---|---|---|---|---|---|---|---|---|---|---|
|
radiation insult in radiation injury to the central nervous system (CNS) is a demyelinating lesion with focal or diffuse areas of white matter necrosis (9).
![]() Figure 19.3 Growth curves of different tissues. (From Tanner JM. Growth at Adolescence. Oxford: Blackwell Scientific, 1962, with permission.) |
(11,12,15, 16, 17). Neurocognitive late effects most commonly follow treatment of malignancies that necessitate CNSdirected therapies, such as cranial radiation, intraventricular or IT or high dose systemic (CNS penetrating) chemotherapy. Thus, children with CNS tumors, head and neck sarcomas, and ALL are most commonly affected. Studies of patients with brain tumors must account for the confounding variables of CNS injury by the tumor, seizures and their therapy, elevated intracranial pressure, and surgery. In patients with leukemia and brain tumors, the use of chemotherapy and the impact of the illness on body image and school attendance may complicate the issue.
RT with cranial RT combined with IT MTX showed that children who were less than 5 years old at the time of treatment and had received RT and IT chemotherapy had lower IQ scores than those who received craniospinal RT alone. Meadows et al. (23) found a significant IQ deficit in children treated with 24-Gy cranial RT combined with IT MTX, as compared with childhood cancer survivors who received no CNS-directed therapy, with the effect greatest among those less than 5 years old. A similar effect on cognition with the addition of IT MTX has been found in children treated for medulloblastoma (26).
places and people associated with the cancer is part of PTSD, the syndrome may interfere with appropriate access to health care. Risk factors include perception of threat to life and severity of treatment, poor family functioning and decreased social support (42, 43, 44, 45, 46, 47).
Table 19.3 Evaluation of Patients at Risk for Late Effects: Central Nervous System | ||||||||||||||||||||||||||||||||||||||||||||||||||||||||||||||||||||||||||
---|---|---|---|---|---|---|---|---|---|---|---|---|---|---|---|---|---|---|---|---|---|---|---|---|---|---|---|---|---|---|---|---|---|---|---|---|---|---|---|---|---|---|---|---|---|---|---|---|---|---|---|---|---|---|---|---|---|---|---|---|---|---|---|---|---|---|---|---|---|---|---|---|---|---|
|
CCSS and compared risk of recurrence, risk of SMN, and risk of death between survivors who did and did not receive treatment with GH. The relative risk of disease recurrence was 0.83 (95% CI 0.37-1.86) for GH-treated survivors. GHtreated subjects were diagnosed with 15 SMNs, all solid tumors, for an overall relative risk of 3.21 (95% CI 1.88-5.46), mainly because of a small excess number of SMNs observed in survivors of acute leukemia. Two other studies have shown no increased risk of relapse or SMN (59,60). The data surrounding SMNs must be interpreted with caution given the small number of events.
triiodothyronine (T3) and thyroxine (T4), are largely bound to plasma proteins when released by the gland. Thyroxinebinding globulin is the major transport protein, and only a small percentage of unbound T3 and T4 is available for activity. The pituitary hormone thyroid-stimulating hormone (TSH) regulates synthesis and release of T3 and T4. TSH secretion is stimulated by the hypothalamic hormone thyrotropin-releasing hormone (TRH) and inhibited by the circulating free thyroid hormones. The pathophysiology of radiation-induced thyroid dysfunction is not precisely defined. Direct radiation damage to the thyroid follicular cells, the thyroid vasculature, or the supporting stroma may occur. Less likely mechanisms that could contribute include the induction by RT of an immunologic reaction or damage from the iodine load administered for lymphangiography. Histopathologic changes in an irradiated thyroid gland include progressive obliteration of the fine vasculature, degeneration of follicular cells and follicles, atrophy of the stroma, and, less commonly, lymphocytic infiltration. Because radiation damage depends on the degree of mitotic activity and the thyroid of a developing child grows in parallel with body growth, this gland might be expected to show an age-related degree of injury and repair.
Table 19.4 Causative Treatment | ||||||||||||||||||||||||||||||||||||||||||||||||||||||||||||||||||||||||||||||||||||||||
---|---|---|---|---|---|---|---|---|---|---|---|---|---|---|---|---|---|---|---|---|---|---|---|---|---|---|---|---|---|---|---|---|---|---|---|---|---|---|---|---|---|---|---|---|---|---|---|---|---|---|---|---|---|---|---|---|---|---|---|---|---|---|---|---|---|---|---|---|---|---|---|---|---|---|---|---|---|---|---|---|---|---|---|---|---|---|---|---|
|
3-5 years after diagnosis. In the study of ALL survivors from the CCSS, the 15-year cumulative incidence was 0.6%. Craniospinal RT was associated with an increased risk of subsequent hyperthyroidism (HR 6.1) compared with chemotherapy alone. In radiation dosimetry analysis, pituitary doses ≥20 Gy combined with thyroid doses ≥15 Gy were associated with hyperthyroidism (75).
series by Paulino et al. (83), in a group of 42 children treated for the Wilms tumor from 1968 to 1994, 7 developed muscular hypoplasia, 5 were found to have limb length inequality, 3 had kyphosis, and another 3 had iliac wing hypoplasia. Scoliosis was seen in 18, with only 1 patient needing orthopedic intervention. Median time to development of scoliosis was 102 months (range 16-146). A clear dose-response relationship was seen, with children treated with lower dosages (less than 24 Gy) having a significantly lower incidence of scoliosis than those who received more than 24 Gy. There was also a suggestion that the incidence was lower in patients who received 10-12 Gy, the dosages currently used for the Wilms tumor, although the sample size was small.
Table 19.5 Evaluation of Patients at Risk for Late Effects: Thyroid | |||||||||||||||||||||||||||||||||||||||||||||||||||||
---|---|---|---|---|---|---|---|---|---|---|---|---|---|---|---|---|---|---|---|---|---|---|---|---|---|---|---|---|---|---|---|---|---|---|---|---|---|---|---|---|---|---|---|---|---|---|---|---|---|---|---|---|---|
|
![]() Figure 19.9 The relationship of radiation dosage and age in the production of slipped capital femoral plates (blue circles). The green circles are other reported cases. Refer to Fig. 2 of Ref. 84 from which data were obtained. Normal epiphyseal plates are represented by orange circles. (From Silverman CL, Thomas PR, McAlister WH, et al. Slipped femoral capital epiphysis in irradiated children: dose, volume, and age relationships. Int J Radiat Oncol Biol Phys. 1981;7:1357-1363, with permission). |
continuous use of dexamethasone. Genetic predisposition may play a role. In an analysis from the Children’s Oncology Group, a PAI-1 polymorphism (rs6092) was associated with risk of osteonecrosis in univariate and multivariate analyses (adjusting for gender, age, and treatment arm) (87). Osteonecrosis is often multiarticular and bilateral, affecting weight-bearing joints predominantly (88, 89, 90, 91).
treatment for childhood cancer. Review of data from the CCSS shows significant cardiovascular morbidity across a number of childhood cancer survivor diagnostic groups, with the caveat that the data are based on self-report. Gurney et al (105). identified that 18% of childhood brain tumor survivors reported a heart or circulatory late effect. Risk was highest among those treated with surgery, RT, and chemotherapy compared to surgery and RT alone, suggesting a potential additive vascular injury from chemotherapy. Among ALL survivors in the CCSS cohort reporting a chronic medical condition, the risk of reporting a cardiac condition was nearly sevenfold higher compared to the siblings. No significant association was identified based on radiation exposure. A similar analysis among acute myeloid leukemia (AML) survivors in the cohort found the 20-year cumulative incidence of cardiac disease to be 4.7. Twenty-one percent of rhabdomyosarcoma survivors reported ≥1 cardiac sequelae compared to siblings. Among survivors of non-Hodgkin lymphoma (NHL), the standardized mortality ratio (SMR) for cardiac disease was 6.9 (110). A recent follow-up study of Wilms tumor survivors reported a cumulative risk of congestive heart failure of 4.4% at 20 years for those who received doxorubicin as part of their initial therapy, and 17.4% at 20 years where doxorubicin was received as part of therapy for relapsed disease. Risk factors for congestive heart failure in this cohort included female sex, lung irradiation with dosages 20 Gy or higher, left-sided abdominal irradiation, and doxorubicin dosage of 300 mg/m2 or more (111). Finally, cardiac complications after BMT may occur, with arrhythmias, pericarditis, and myopathies predominating. High-dose cyclophosphamide is clearly a causative agent. TBI is a secondary contributing factor.
Table 19.6 Evaluation of Patients at Risk for Late Effects: Musculoskeletal | ||||||||||||||||||||||||||||||||||||||||||||||||||||||||||||||||||||||||||||||||||||||
---|---|---|---|---|---|---|---|---|---|---|---|---|---|---|---|---|---|---|---|---|---|---|---|---|---|---|---|---|---|---|---|---|---|---|---|---|---|---|---|---|---|---|---|---|---|---|---|---|---|---|---|---|---|---|---|---|---|---|---|---|---|---|---|---|---|---|---|---|---|---|---|---|---|---|---|---|---|---|---|---|---|---|---|---|---|---|
|
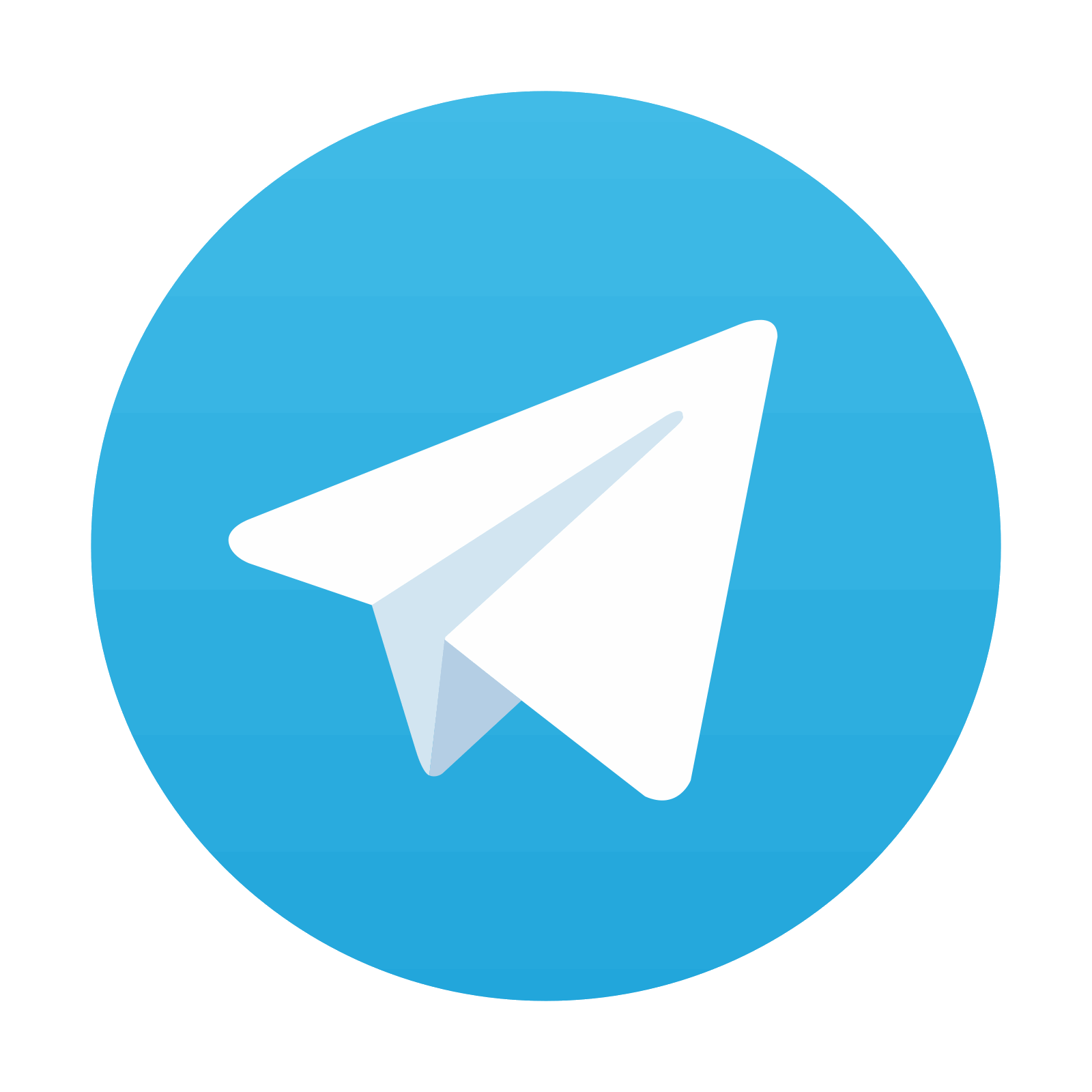
Stay updated, free articles. Join our Telegram channel
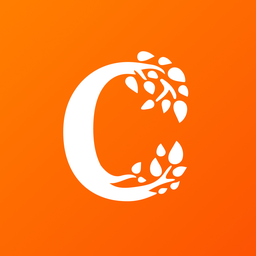
Full access? Get Clinical Tree
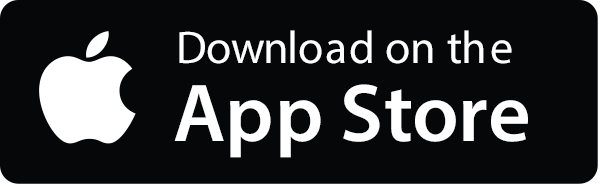
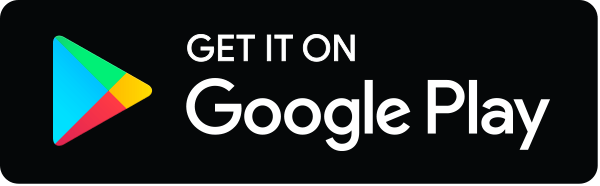
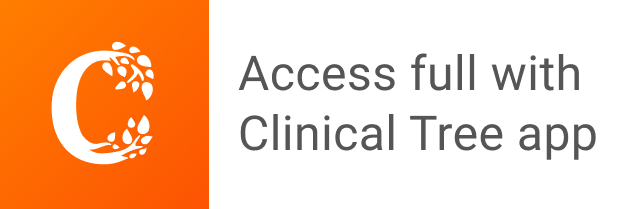