Lung
David A. Bush
Despite intensive research into new treatment strategies, lung cancer continues to be a major health problem worldwide and remains the most frequent cause of cancer death in North America.1 Non-small cell lung cancer is the most frequently diagnosed histologic type of lung cancer and carries a poor prognosis despite intensive therapies. The only subgroup that carries a better than 50% long-term survival is patients diagnosed with early-stage disease; that is, peripherally located T1 or T2 tumors without evidence of nodal or distant metastases. Surgical resection in such patients can produce 5-year survival rates of approximately 60% or 70%.2
Unfortunately, only approximately 15% of patients will be diagnosed with disease at this stage. The largest group is patients presenting with stage III disease, commonly referred to as locally advanced non-small cell lung cancer. These patients generally have involvement of the chest wall, mediastinum, and/or mediastinal lymph nodes, any one of which usually precludes surgical resection. These patients are managed with a combination of chemotherapy and radiotherapy as the primary modality for local-regional disease control within the chest.
The goal of definitive thoracic radiotherapy in patients with non-small cell lung cancer is to reliably eradicate intrathoracic disease while respecting the tolerances of nearby normal structures. This goal certainly has not yet been achieved. Various radiation techniques have also shown some evidence of incremental improvement, such as hyperfractionation, accelerated fractionation, conformal dose delivery techniques, and dose escalation.3, 4, 5, 6 Most innovative radiation treatment techniques have focused on conformal radiation treatment delivery with three-dimensional (3-D) treatment planning and, in some cases, intensity-modulated radiation therapy (IMRT).7,8 The goal of each of these treatments is to deliver higher doses to target regions in an effort to improve local tumor control within the constraints of surrounding normal tissue regions such as heart, lung, esophagus, and spinal cord.
Proton beams and heavier charged particles possess physical characteristics that allow radiation doses to be more accurately deposited within target regions while minimizing dose to the surrounding nontarget tissues. These properties may allow safe dose escalation in patients with non-small cell lung cancer. Because local tumor control is insufficient with conventional treatment and because there is evidence that higher doses may improve tumor control, there seems to be a rationale for the use of proton radiation therapy (RT) in patients with lung cancer. Fowler analyzed the potential use of proton beam treatment in lung cancer cases and, using biological modeling, estimated that significant improvements in local tumor control and survival may be possible.9 Because of the normal tissue-sparing properties of proton beams, this may be achievable without increased normal tissue complications and possibly may even decrease the severity of toxicity seen in comparable treatments with photons. This is the basis for multiple clinical investigations into the use of charged particle therapy in patients with lung cancer, being conducted at centers around the world.
TREATMENT PLANNING COMPARISONS
As discussed elsewhere in this text, proton beams and heavier charged particles all exhibit a Bragg peak with normal tissue sparing beyond the peak not possible with photon beams. While it might seem self-evident that charged particle beams will always be superior to photon beams for targeted cancer treatment, special dosimetric circumstances exist for lung cancer treatment that differ from charged particle therapy elsewhere in the body. Tumors of the lung are invariably surrounded by aerated pulmonary parenchyma, in which an essentially water density equivalent tumor is
surrounded by substantially less-dense lung tissue. Aerated lung tissue has reduced stopping power compared to other soft tissues of the body, which affects the stopping distance or distal edge of a spread-out Bragg peak. This can cause charged particle beams to travel some distance beyond the distal edge of the target volume, which is not typically seen in tumors located in other parts of the body. Despite this shortcoming, there are still reasons to believe that the dosimetry provided by charged particle beams is an improvement over that with photon beams.
surrounded by substantially less-dense lung tissue. Aerated lung tissue has reduced stopping power compared to other soft tissues of the body, which affects the stopping distance or distal edge of a spread-out Bragg peak. This can cause charged particle beams to travel some distance beyond the distal edge of the target volume, which is not typically seen in tumors located in other parts of the body. Despite this shortcoming, there are still reasons to believe that the dosimetry provided by charged particle beams is an improvement over that with photon beams.
A formal treatment planning comparison between proton and photon treatment planning has been published by Chang et al.10 Their analysis of 10 patients with medically inoperable stage I lung cancer compared proton treatment plans to 3-D conformal photon plans at two different dose levels (66 Gy and 87.5 Gy). Analysis of these plans revealed approximately 50% reduction in nontarget lung dose when protons where used. The normal lung tissue-sparing effect seems to be increased with the high-dose plans, indicating an additional benefit for dose escalation when proton beams are used. Fifteen patients with stage III lung cancer were also analyzed, comparing 3-D photon, IMRT, and proton beam plans at two different doses (63 Gy and 74 Gy). Again results showed a substantial reduction in the nontarget lung dose, and again, the proton benefit seemed to be more evident with the higher dose plans. Doses to the heart, esophagus, and spinal cord were also found to be significantly reduced. This study appears to indicate that proton beams may be able to achieve target higher doses with more significant normal tissue sparing than 3-D conformal RT or IMRT.
DOSIMETRIC CONSIDERATIONS
Whenever targets within the lung parenchyma are selected for treatment, consideration must be given to physiologic internal target motion. Physiologic tumor motion within the lung has been well described in the medical literature. Lung tumors typically show the greatest motion in the superior/inferior dimension, and movement is highly dependent on the region of the lung wherein the tumor resides, that is, tumors near the diaphragm will show the largest respiratory excursion. The simplest method to account for internal target motion is to measure the 3-D excursion of the tumor and expand the treatment volume to encompass an area within the lung that covers all possible tumor positions. When small tumors are treated with motion parameters of <1 to 2 cm, this method is likely adequate. This technique has been used for patients with peripheral lung tumors of 1 to 4 cm in size at Loma Linda University Medical Center (LLUMC) for the last 10 years, with an excellent safety profile with an exceedingly low incidence of radiation pneumonitis.11
For larger tumors, and when motion parameters are larger, it is likely that some sort of beam gating or respiratory control may significantly reduce dose to normal lung tissue. Several techniques have been described for use with photon radiotherapy and/or IMRT. These solutions also apply to proton or other heavy charged particle radiotherapy, although special considerations do apply. Respiratory monitoring with beam gating requires integration into the beam control system and does increase treatment times, but these challenges have been successfully addressed at several proton and carbon ion facilities. Patients may also be requested to follow a reproducible breath-holding or shallow breathing technique that can be used to physiologically suspend tumor motion during treatment. This may increase the beam-on time compared to the previously described volume expansion technique; however, patients with compromised pulmonary function will likely have difficulty performing these techniques potentially creating compliance problems. A third method would be to use an external visualization of internal targets as they move in real time, with integration to a patient position device that will move the patient during treatment in a way that allows the target to remain still relative to the treatment beam. Such a system has been described for use with photon radiation and seems to provide a solution that maximizes beam-on time as well as patient compliance. If such a system were used with charged-particle beams, there are other considerations that may be important. Changing densities of the chest wall (ribs and soft tissues) moving relative to the stationary treatment beam would create varying particle path lengths and uncertain beam stopping. Such uncertainties may compromise full treatment of the targeted area. It is possible, however, that such variances may be considered in the planning process (i.e., compensator smearing) to allow full coverage of the target region at all times. A report by Engelsman compared computerized treatment plans using various techniques to account for respiratory motion in planning proton treatments.12 Target expansion using 4-D treatment planning in which the planning computed tomography (CT) data set includes multiple phases of the respiratory cycle was found to provide the most reliable target coverage. While there are as yet no reports comparing clinical outcomes of these different approaches and the ideal method for use with charged particle beams remains undefined, experience with respiratory gating systems is being accumulated and studied in a number of the charged particle facilities around the world including Tsukuba University and National Institute of Radiological Sciences (NIRS) (Chiba) in Japan and the Francis H. Burr Proton Therapy Center at Massachusetts General Hospital (MGH) in Boston.
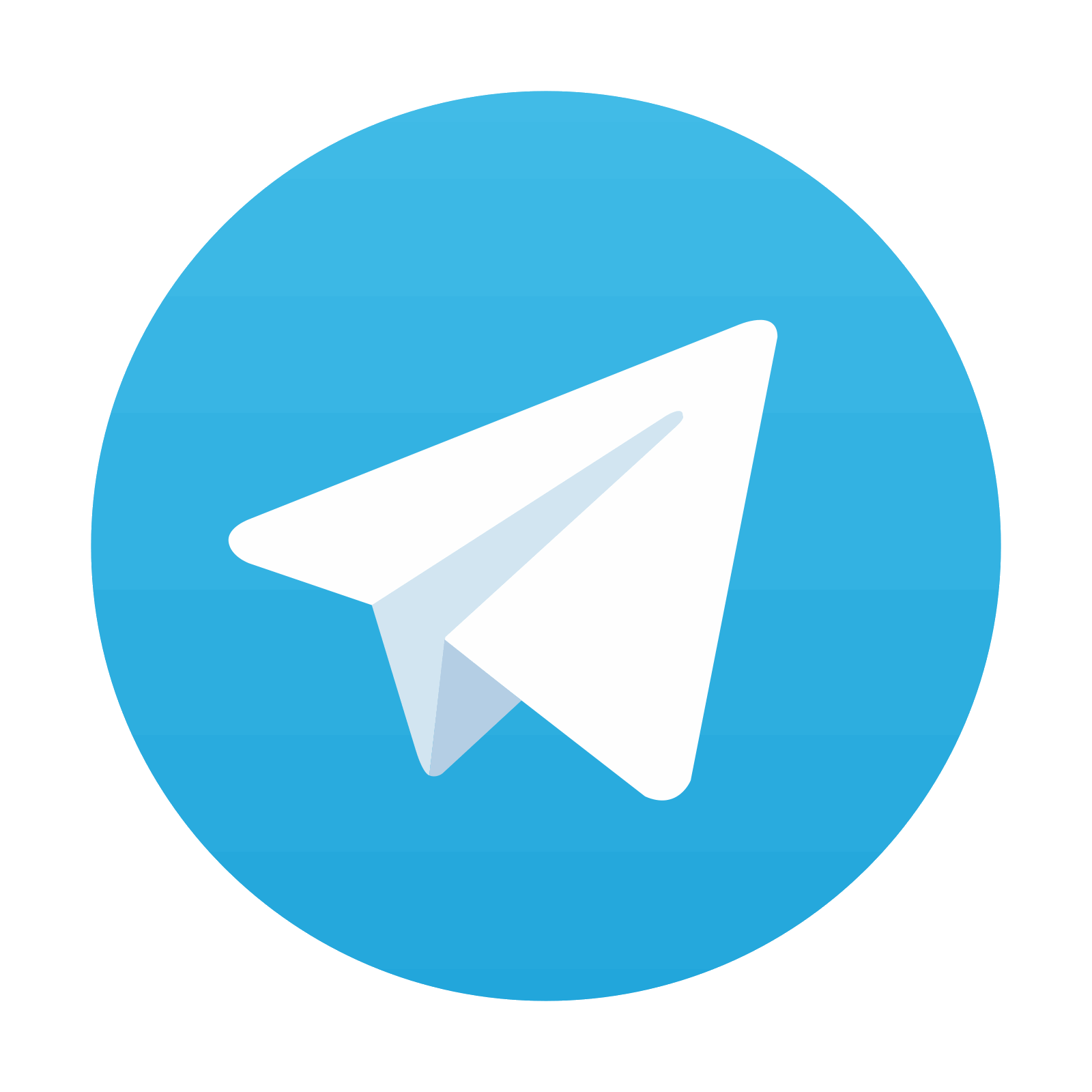
Stay updated, free articles. Join our Telegram channel
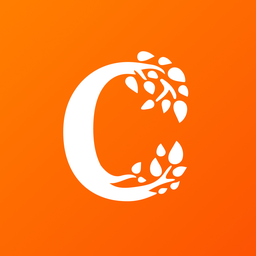
Full access? Get Clinical Tree
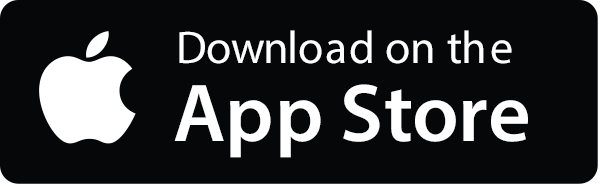
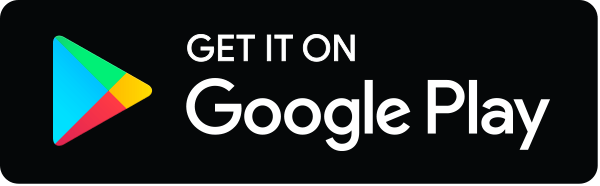