Jacob Geleijns, Denis Tack Medical imaging with X-rays, radionuclides, magnetic resonance imaging (MRI) and ultrasound is increasingly utilised in health care for screening, diagnosis and follow-up of disease. Imaging techniques using X-rays or radionuclides have become more sophisticated and, advanced 3-dimensional imaging and 4-dimensional dynamic imaging with computed tomography (CT), single-photon emission computed tomography (SPECT) and positron emission tomography (PET) have become widely available and are frequently used. As a consequence, the radiation exposure of the Western population increased and simultaneously concerns about radiation risks grew. The relevance of imaging with ionising radiation in medicine is widely acknowledged due to the benefits of the imaging studies for clinical care of patients. It is, however, important that the users of radiation are aware not only of the clinical benefits of medical imaging but also of the short- as well as long-term radiation risks to their patients and themselves. Soon after the discovery of X-rays and radioactivity, it became evident that high doses of ionising radiation could cause somatic damage to organisms, certain organs and tissues (e.g. erythema), but it was only many years later that late carcinogenic and late non-carcinogenic effects, including genetic effects, from low radiation doses were appreciated. Current knowledge of the risks of ionising radiation is based on a wide range of epidemiological evidence, from animal studies and cell biology. The most significant body of information comes from still ongoing studies of those who survived the atom bombs dropped on Hiroshima and Nagasaki in 1945 (the so-called Life Span Study) and several epidemiological studies on radiation workers and cured radiotherapy patients.1,2 Recently results of an epidemiological study on the exposure from CT imaging in childhood and the subsequent radiation risks of leukaemia and brain tumours have been published. This study is unique since it is the first large epidemiological study on paediatric patients that underwent a modern CT imaging. The risk estimates from this study are broadly consistent with the results from the Life Span Study, thus providing support for the existing cancer risk estimations, particularly for children.3 The detrimental health effects of radiation can be divided into deterministic effects and stochastic effects. Deterministic effects, also called tissue reactions, are associated with radiation-induced killing or malfunction of cells. They are characterised by a threshold dose below which the effect does not occur. Just noticeable lens changes (cataract), transient skin damage (erythema) and transient oligozoospermia are examples of mild deterministic effects. Table 1-1 shows approximate threshold doses for mild deterministic effects and their latency time. The deterministic effects that have been observed in patients after X-ray imaging are skin effects, knowingly erythema and epilation. The threshold skin dose for erythema and epilation is much higher than the doses that patients are usually exposed to. Therefore, the occurrence of deterministic skin effects after diagnostic X-ray imaging is very rare and if it occurs it is generally associated with incorrect use of the imaging equipment. A known example is the occurrence of epilation after diagnostic CT brain perfusion studies due to the use of improper acquisition protocols.4 Mild and transient deterministic radiation effects such as skin erythema and epilation have sometimes been observed after properly performed but lengthy and complex X-ray-guided cardiac and neurological interventions, and after CT-guided interventions. The severity of the deterministic effect increases with dose. Incidents with very high skin doses after X-ray-guided interventions have resulted in serious skin effects such as desquamation, ulceration and necrosis and in some cases treatment with skin grafts was necessary.5,6 Stochastic effects are associated with damage to the DNA of cells and they may result in cancer or heritable effects. Stochastic effects have an impact on the clinical practice of medical imaging with ionising radiation since it is assumed that the incidence of cancer or heritable effects will rise in direct proportion to the dose in organs or tissues. This dose–response model is generally known as ‘linear-non-threshold’ or LNT model. Stochastic effects are of a random statistical nature, meaning that the probability of occurrence of the effect increases with dose. It takes a long time until DNA damage comes to expression. The latency period for induction of leukaemia is at least 2 years, and on average about 8 years; for solid tumours the latency period is at least 10 years, and on average 20 years. This means that radiation risks are relatively small for older patients, since their life expectancy is short compared to the latency period, and in contrast, radiation risks are relatively high for young patients. Radiation damage to germ cells may result in genetic effects in future offspring. To date there is no scientific evidence of radiation-induced hereditary effects in the offspring of humans. However, based on animal experiments, it is assumed that hereditary effects in humans may occur and radiation protection is therefore aimed to avoid any hereditary effects. The dose–response model at low doses has always been a subject of debate. For pragmatic reasons the LNT model is widely used in radiation protection. The LNT model implies that all exposures, even if they are very low, carry a certain risk. Another dose–response model is a linear model with an arbitrary threshold at very low doses, meaning that there is no radiation risk below a certain dose level. It has been also suggested that there may be even some protective effect of radiation at very low doses, while other studies show hyper-radiosensitivity at very low doses (Fig. 1-1). The actual shape of the dose–response model at very low doses remains a matter of still ongoing scientific controversy.7 Table 1-2 shows quantitative estimates risk of cancer incidence and hereditary effects for adult workers and the whole population according to the International Commission on Radiological Protection (IRCP 103). The risks are expressed per unit of effective dose (Sv). A total risk of 4.2 × 10−2 per Sv means that an exposure to 2 mSv (0.002 Sv) is associated with a risk of 4.2 × 10−2 × 0.002 = 0.00008; or in other words a risk of 1 in 10,000. Risks are slightly higher when they are averaged for the whole population compared to the working population due to the consideration of children. Young children are about a factor of three more sensitive to radiation effects than the average population. At higher ages the sensitivity for radiation effects drops quickly; radiation risks become smaller than those for the population average at the age of 30 and higher. TABLE 1-2 Cancer Incidence Weighted for Lethality and Life Impairment and Hereditary Effects in Offspring for Adult Workers and the Whole Population Source: ICRP 103. International Commission on Radiological Protection. Deterministic and stochastic effects may occur as a result of in utero radiation and it is recognised that the fetus is highly radiosensitive during prenatal development. The most vulnerable period for deterministic effects is between the 2nd and 20th week of gestation. In normal circumstances, there is no risk for deterministic effects, like malformation, growth retardation, mental retardation and death associated with diagnostic medical imaging. No deterministic effects are expected to occur at a (cumulative) fetal dose below 100 mGy, and clinical (cumulative) fetal exposures in diagnostic imaging are expected to remain well below 100 mGy. Like in adults, a small stochastic effect of radiation-induced cancer exists after fetal exposure. The cancer risk of radiation exposure in utero is on the same order of magnitude as the risk of exposure during childhood; i.e. as stated earlier, about three times higher than that of the whole population. The European Union requires from its member states that they implement legislation that ensures basic safety standards for appropriate protection against ionising radiation, including protection against radiation that is used for medical diagnosis. European basic safety standards were put into force in a council directive in 1996 and a proposal for new European basic safety standards was published in 2012. General legislation covers the protection of people exposed to radiation within their professional activities like radiologists and radiographers. More specific legislation with regard to diagnostic imaging using ionising radiation regulates the justification and optimisation of the medical exposures; the latter includes appropriate referral for medical imaging; certified training on radiation protection of involved professionals; and the quality and safety of the imaging equipment. European legislation on radiation protection also requires that clinical audits are implemented in accordance with national procedures. In those clinical audits, aspects of local practice are assessed against ‘good practice’. Various methods are being used for clinical audit, and the European Commission published guidelines on clinical audit for medical radiological practices.8 The aim of radiation protection against ionising radiation in diagnostic medical imaging is to restrict radiation dose to staff, patients and the general public to remain below the level at which deterministic effects occur and the probability of stochastic effects is limited to an acceptably low level. To achieve this, the ICRP recommends the application of three principles: justification, optimisation and limitation.1 Justification implies that no practice resulting in exposure to ionising radiation should be adopted unless it results in sufficient net benefit to exposed individuals or society to offset the detriment. The radiation exposure resulting from new applications of diagnostic imaging with ionising radiation should be justified in advance, and the justification of existing practices should be reviewed regularly. Justification of diagnostic medical imaging applies to patients and to professionals working with radiation. Special conditions refer to the following situations: the justification of population screening like mammography screening, the justification of radiation exposure of volunteers in biomedical research9 and the exposure of carers and comforters. Carers and comforters are individuals who are exposed to ionising radiation because they support and comfort another person while undergoing medical exposure, e.g. a mother that is exposed to scattered radiation while supporting her child during an X-ray examination. It is expected from physicians that any referral of patients to medical imaging involving X-rays is justified by the fact that this examination will contribute to ‘good medical care’. It should be carefully outweighed between the medical information that is needed, the information that can be retrieved and the type of medical imaging chosen and whether it involves or does not involve radiation. Guidance for proper referrals are published by, for example, European Commissions as referral guidelines for imaging for healthcare professionals who prescribe imaging investigations involving ionising radiation.10 Guidelines for proper use of medical imaging are also provided by professional bodies on an international level, for example by the European Society of Radiology, as well as on a national level. Optimisation requires that the likelihood of incurring exposure, the number of people exposed and the magnitude of their individual doses should all be kept as low as reasonably achievable (ALARA). In diagnostic imaging, the optimisation usually means finding a balance between the required image quality and the associated radiation exposure. The radiologist, nuclear medicine specialist or radiographer carrying out any practical aspect of medical imaging with ionising radiation has an important role in keeping the dose to the patient as low as reasonably practicable commensurate with the diagnostic purpose. The type of examination and imaging technique must be selected so as to minimise the dose to the patient. Measures for achieving this are, for example, to limit the number of views in radiography, to limit the number of series in CT, and to keep fluoroscopy time as short as possible. Optimisation also implies that equipment for medical imaging is initially selected so as to be as dose-efficient as possible and is installed and correctly maintained thereafter. A medical physicist can help to achieve this; in addition the medical physicist should be involved in the optimisation process, in patient dosimetry, quality assurance and any efforts for radiation protection in medical exposures. Other examples of optimisation are the implementation of specific acquisition protocols for CT examinations of obese and slim patients or for young children. In nuclear medicine, optimisation can be achieved by carefully determining the required activity. Optimisation of radiation protection of professionals can be achieved by wearing the most appropriate protective clothing (lead apron, thyroid shield) and the use of extra devices for shielding like lead curtains and lead shields. Limitation means that the total dose to any individual in a planned exposure situation should not exceed the dose limits specified by the legal authorities. This is to ensure that no individual is exposed to an unacceptable radiation risk. Dose limits also apply to professionals like radiologists, nuclear medicine physicians and radiographers. A patient, while not undergoing a radiological procedure, is considered a member of the general public. Most countries adopt in their legislation the dose limits recommended by the ICRP in publication ICRP 103 (Table 1-3). Recently the ICRP revised its recommendation for the dose limit for the eye lens. Based on recent epidemiological evidence, the ICRP reduced in 2011 its recommended dose limit for occupational exposures of the eye lens from 150 to 20 mSv per year according to ICRP publication 118.11 This may have consequences, particularly for interventional radiologists. TABLE 1-3 The ICRP Recommended Dose Limits per Year in Planned Exposure Situations1,11 Field studies in different hospitals have shown that there is a wide range of doses delivered for the same type of investigation. Therefore, although absolute dose limits are not appropriate, European legislation requires that so-called ‘diagnostic reference levels’ (DRLs) must be established.12 The diagnostic reference level (DRL) refers to the dose value that should not be exceeded during a routine examination of a patient with a normal posture. Patient doses should be audited regularly and trigger immediate action to reduce them whenever DRLs are exceeded. The concept of diagnostic reference level has its origins in the early 1990s in the United Kingdom. Introduction of DRLs seemed to help to ensure that in the course of several years, the radiation exposure in radiological studies in the United Kingdom actually declined. Good practice implies that the different professional groups that are responsible for radiation protection work together in a Radiation Protection Committee consisting of representatives both from management and staff. The committee meets regularly to review implementation of the regulations, to evaluate staff doses and to discuss any incidents. In medical imaging using ionising radiation there is always a trade-off between the radiation risk for the patient and the image quality of the study. Better image quality is generally associated with a higher radiation exposure. The radiation exposure of a plain chest radiograph is, for example, much less compared to a volumetric CT examination of the chest. Image quality of radiographs, fluoroscopy and CT can generally be improved by using a higher dose. For example, for a radiograph a fourfold increase in dose would be required to achieve a noise reduction of 50%. In nuclear medicine, the administration of more radionuclide activity may be used to achieve better image quality. Whenever using X-rays or radionuclides, the technique should be selected so that the image quality is tailored to the required diagnostic information. The risk associated with a particular radiological examination depends on the sensitivity of the exposed organs and tissues and on the average dose received by each of them. It is common practice to derive the effective dose from a large number of average organ and tissue doses. The calculation of effective dose is based on the tissue weighting factors that were published by the ICRP.1 The tissue weighing factors are proportional to the sensitivity for radiation-induced cancer and hereditary effects. The trunk with organs such as colon, lung, stomach and breasts is more sensitive to radiation exposure than the head. The extremities are particularly insensitive for radiation exposure. Direct measurement of organ and tissue doses or effective dose is not possible, but several indirect methods are available for the assessment of effective dose. Almost all X-ray units provide the user with an indication of patient dose. The dosimetric quantity that is used depends on the type of examination. In radiography and fluoroscopy it is common practice to use the dose–area product (DAP). In CT the computed tomography dose index (CTDI), and the dose–length product (DLP) are used. These operational dose qualities are often stored together with patient information in the digital archives like PACS and thus the radiation exposure of individual patients may be retrieved afterwards. The dose–area product is measured during the X-ray examination with a large, flat-plate ionisation chamber attached to the collimator on the X-ray tube. The DAP meter measures the product of the average dose within a trans-section of the X-ray beam tube and the corresponding area of the X-ray beam. DAP provides a good indication of patient dose. Indications of patient dose in CT are based on measurements made with a pencil ionisation chamber in standard CT phantoms.13,14 A 16-cm diameter polymethyl methacrylate (PMMA) phantom is used for the dosimetry of CT head imaging and a 32-cm diameter phantom for CT of the body. Software of the CT device calculates the CTDI and the DLP from the acquisition parameters for each clinical CT image. Dosimetry in mammography is complicated and it is usually performed by a medical physicist. In mammography entrance dose is measured using a Perspex phantom and the mean glandular breast dose is calculated from the measurements.15,16 Some mammography units provide the user with an indication of the mean glandular dose after each clinical examination. Effective dose for radiography and CT can be assessed by conversion factors; some examples are provided in Table 1-4. TABLE 1-4 Effective Dose Conversion Factors for Radiography and CT of the Average Adult Patient In radionuclide imaging, the effective dose for the patient depends on the radionuclide and its activity. Its chemical form determines the distribution of the radionuclide within the body and its metabolic rate. The effective dose may also depend on individual patient physiology; for example, if kidney function is impaired, clearance may be slow. Patient doses received in various common radionuclide examinations and the means to calculate them are generally based on the ICRP publications 80 and 106;17,18 these publications cover most of the pharmaceuticals in current use in diagnostic nuclear medicine. Regular assessment of patient dose is carried out in European countries, and in most countries this is coordinated by one institute that collects doses throughout the country and subsequently publishes national patient dose reviews. Such national overviews of patient dose may facilitate the establishment of national diagnostic reference levels for certain examinations. An overview of typical effective doses resulting from diagnostic imaging are provided in Table 1-5 (radiography and CT) and Table 1-6 (nuclear medicine, including the effective dose conversion factor). CT and nuclear medicine give rise to the highest effective dose; doses for radiographs are much lower. Effective dose is negligible for radiography of extremities and for dental radiographs. TABLE 1-5 Typical Effective Doses for Digital Radiography and for CT of the Average Adult Patient In diagnostic medical imaging, images are nowadays almost exclusively acquired in digital technique, including dental applications, radiography, mammography, nuclear medicine and computed tomography. X-ray films and delicate film processing is a thing of the past. The imaging equipment generally acquires raw digital images that must be optimised by image (pre)processing. Digital images are displayed on a workstation that offers applications for further image processing and advanced visualisation. Images can be transferred to, archived in and retrieved from a PACS system. An advantage of digital systems is the wide dynamic range that makes it possible to display structures with wide attenuation differences equally well and to avoid under- or overexposure, that way reducing the need for repeat exposures. Since with digital systems image density and contrast are automatically optimised, acquisition dose cannot be controlled visually any more. A pitfall of digital systems is therefore that the exposure control of the system is set in a way that too high doses occur systematically. It is therefore important that the technicians are aware of the doses actually being applied by recording and evaluating the dose readings from the systems. In radiography there are two distinct systems, commonly known as computed radiography (CR) and direct radiography (DR). CR is a cassette-based system and so can be used with existing X-ray equipment. Storage phosphor imaging plates are contained within cassettes. After exposure the storage phosphor image plate stores the absorption as a pattern of excited electrons. The plate is subsequently ‘read-out’ by using a laser beam to extract the stored image information as a raw digital image. Direct radiography systems use solid-state flat panel detectors. There are two different types that use either direct or indirect conversion of the X-ray photons into electrical charge. Indirect means that the X-ray image is converted into light using, for example, a caesium iodide scintillator. Subsequently, by using a matrix of photodiodes this light is converted into electrical charge and into a raw digital image. In the direct system, X-rays interact with an amorphous selenium plate which converts the X-ray photons image directly into an electrical signal. Digital fluoroscopy can be performed with an image intensifier but also in fluoroscopy more and more digital flat panel detectors are used. The detector technology of photon counting is currently available solely for clinical mammography but might become available also for other applications in the future. Photon counting systems have the advantage of low electronic noise and the possibility of measuring the energy of each detected X-ray photon. Photon-counting detectors are relatively small and for full-field coverage the detector is used in an imaging mode. The energy response of digital systems must be taken into account when establishing image acquisition protocols, and has led to a tendency to use higher tube voltage in mammography and to use lower voltage in chest radiography. It is generally acknowledged that digital radiography and digital fluoroscopy techniques offer substantial improvement of image quality compared with analogue technique-like film-screen radiography. ICRP Publication 93 gives a review of the dose issues involved in digital radiology.19 Manufacturers are constantly improving X-ray equipment in order to produce the best image at the lowest dose possible. Features of modern X-ray systems are, for example, carbon fibre and other low-attenuation materials in table tops and grids, high-frequency generators and additional beam filters to reduce entrance skin dose. In fluoroscopy last image hold and road mapping, pulsed fluoroscopy, optimised presets for automatic control of kV and mA for different procedures, virtual field indicators and collimators and lasers for beam centring are techniques for optimising image quality and lowering the dose. Special attention is required for optimising patient dose in computed tomography. The rapid increase of the number of CT examinations worldwide made it the biggest contributor to the collective dose from X-ray examinations. CT is also used in hybrid systems like SPECT-CT and PET-CT. CT systems may be equipped with multiple dose-reducing features such as automatic exposure control which modulates the tube current to account for variations in patient attenuation and dynamic collimators that reduce the over-ranging at the start and the end of the CT imaging. Advanced algorithms for noise reduction and iterative reconstruction techniques may help to reduce patient dose in CT even further. ICRP Publication 87 provides further information on factors affecting dose in CT.20 There is some misconception about in-plane bismuth shielding of the breast, thyroid and eye lens in CT. In-plane bismuth shielding is counterproductive and should not be applied.21–23 Small CT systems constructed of relatively simple components are often referred to as cone beam CT systems. They are used, for example, for dental applications. The detector of these cone beam CT systems is similar to that of digital radiography systems, and the performance of the detector is often poorer than the detectors used in the regular CT machines. As a consequence, the low tissue contrast of cone beam CT systems is poor but cone beam CT may offer good spatial resolution. In nuclear medicine patient dose is dependent on the activity administered. Standard operating procedures should specify the normal activity to be used in adult patients and the imaging protocol to be used (e.g. choice of collimator). Generally, dynamic and tomographic studies require more activity to be administered. Adequate patient preparation is essential for the procedure to be effective; taking of fluids should be encouraged to increase the clearance rate from the body and thus reduce the dose received. If a patient requires multiple procedures, some of which involve radioactive materials, the order in which the tests are done is important to avoid one test interfering with another. Dual-headed cameras are now common and these are capable of automatically acquiring whole-body images in one pass, allowing the simultaneous collection of two or more images (e.g. anterior and posterior or lateral or oblique views). They have reduced the acquisition time for many studies compared to single-headed cameras, including SPECT sequences, thus reducing the chance of patient movement and so improving image quality. PET is becoming more common and its use, especially in oncology, is well recognised. For the patient, the radiation dose received using a dedicated PET system is slightly higher than conventional nuclear medicine procedures. A more recent development in nuclear imaging is to combine a SPECT or PET system with a CT system in order to give more precise anatomical location of the activity and attenuation correction. The patient thus receives a dose for both procedures, but often the CT imaging can be performed at a very low dose. Special attention for radiation protection is required in specific areas involving pregnant patients, paediatric patients, research, population screening and procedures with high doses. The possibility of pregnancy should be taken into account in women of child-bearing age for any radiological examination where the fetus may be in the primary beam or very close to it and any nuclear medicine investigation. If the patient is pregnant or is probably pregnant, justification for the procedure should be reviewed extra carefully. In addition, before performing examinations of the abdominal or pelvic region, the operator should specifically interrogate before giving the exposure whether the patient may be pregnant. It is also advisory to post notices at several places within the department requesting patients to notify staff if they think they may be pregnant. If, despite the patient being pregnant, the exposure cannot be delayed, special attention should be given to optimisation of the exposure, taking into account the exposure of both the expectant mother and the unborn child. In nuclear medicine, for radionuclides that are rapidly eliminated by the bladder, frequent voiding should be encouraged to reduce fetal dose since the bladder acts as a reservoir of activity. ICRP Publication 88 gives doses to the fetus from intakes of radionuclide by the mother.24 A common clinical indication of diagnostic imaging of a pregnant patient is a suspected acute pulmonary embolism. Imaging can be done either by performing a perfusion scintigram (mostly without corresponding ventilation scintigram and with a lower dose of radionuclide) or CT. There has been an extensive discussion also in the literature about which examination is preferable, considering that a CTA causes a lower fetal dose but a higher maternal dose to the breasts than the nuclear medicine procedure.25 A summary of fetal doses from a range of common diagnostic procedures is given in Table 1-7. It must be noted that at no point exposure of the fetus to diagnostic levels of radiation represents, in itself, a reason to terminate a pregnancy, but efforts should be made to avoid or minimise the irradiation of known pregnant or potentially pregnant women. As children are more radiosensitive than adults and their longer life expectancy gives greater opportunity for the radiation detriment to be expressed, special care must be taken to ensure that any radiation doses to children are justified by the diagnostic provided by the procedure. In addition, very young children may be uncooperative and need some form of sedation or immobilisation. The assistance of a parent will usually help to increase the cooperation of the child, but the parent should be provided with protective clothing and the possibility of a mother being pregnant must be considered. The use of a grid is often unnecessary, particularly with smaller children, and removing it can reduce the dose by as much as 50%. The X-ray beam should be well collimated and appropriately sized and gonad shielding could be used to minimise the dose to organs outside the area of interest. In nuclear medicine procedures, the biological distribution, uptake and retention of radiopharmaceuticals vary considerably throughout childhood. The activity administered should be the minimum consistent with obtaining a diagnostic result. For a child, the adult-administered activity in simple proportion to the smaller body weight (as long as the activity is not too low) will usually produce an image quality and imaging time comparable with that expected for adults.
Medical Physics
Radiation Risks
Radiation Risks
Deterministic and Stochastic Effects
Effect
Risk (10−2 Sv−1)
adult workers
whole population
Cancer
4.1
5.5
Severe hereditary effects
0.1
0.2
Total
4.2
5.7
Fetal Exposure
Legislation and Principles of Radiation Protection
Type of Dose Limit
Occupational Exposures (mSv per Year)
Exposure of the General Public (mSv per Year)
Effective dose
20
1
Lens of the eye
150/20*
15
Skin
500
50
Hands and feet
500
Not applicable
Patient Doses in Diagnostic Imaging
Estimating Patient Doses
Radiography, Adult
Effective Dose Conversion Factor (mSv/Gy.cm2)
Chest PA
0.26
Chest LAT
0.17
Abdomen AP
0.24
Pelvis AP
0.24
Skull PA
0.06
Cervical spine AP
0.28
Cervical spine LAT
0.08
Thoracic spine AP
0.24
Thoracic spine LAT
0.12
Lumbar spine AP
0.27
Lumbar spine LAT
0.13
CT, Adult
Effective Dose Conversion Factor (mSv/mGy.cm)
Head
0.0019
Neck
0.0051
Chest
0.015
Abdomen
0.015
Pelvis
0.013
Typical Patient Doses
Radiography, Adult
Effective Dose (mSv)
Chest PA
0.02
Chest LAT
0.04
Abdomen AP
0.45
Pelvis AP
0.30
Skull PA
0.015
Cervical spine AP
0.02
Cervical spine LAT
0.005
Thoracic spine AP
0.2
Thoracic spine LAT
0.15
Lumbar spine AP
0.30
Lumbar spine LAT
0.25
CT Scan, Adult
Effective Dose (mSv)
Head
1–2
Chest
4–6
Abdomen
8–12
Detectors for Radiography and Fluoroscopy
Optimising Patient Dose
Optimising Patient Dose in Nuclear Medicine
Areas of Special Attention
Pregnancy and Potential Pregnancy
Infants and Children
Stay updated, free articles. Join our Telegram channel
Full access? Get Clinical Tree
Medical Physics
Chapter 1
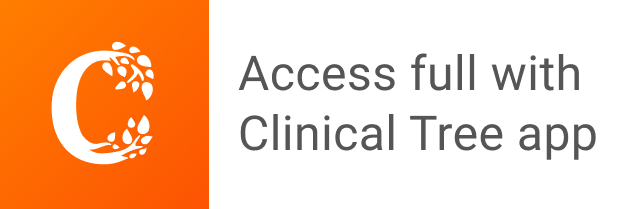