Fig. 1
Resting state MEG for alpha-band oscillatory activity in healthy children and children “at risk” for OCD age 6–12 y (Ciesielski et al. 2013, study in progress)
Furthermore, the developmental changes, in both gray and white matter, may be captured by measuring MEG latency in task-related networks. Based on recent studies, it is suggested that nesting oscillations by phase or amplitude of a lower frequency (theta, 3–7 Hz, or alpha, 8–13 Hz) may modulate phase or amplitude of a higher frequency (beta, 13–30 or gamma, 30–60 Hz). It is also suggested that phase-amplitude coupling interacts with local and long-range functional connectivity to integrate information across-networks. A study, employing perception of emotional and neutral faces, has reported reduced local and long-range functional connectivity in autism-spectrum disorders using this approach [Khan et al. 2013].
In summary, although resting-state fMRI studies have provided consistent evidence of correlated changes in spontaneous neural activity within long-range networks, fMRI allows only indirect evidence of brain connectivity. MEG, however, measures the ongoing physiological neural activity in vivo. Because of the high temporal resolution and measurement of specific spectral frequencies, MEG (and EEG) is uniquely suited for measurements of connectivity of groups of neurons within a small envelope of time, thus providing valuable insight into the rapid and complex changes in developing networks.
3 Developmental MEG Studies of Long-Range Networks
3.1 MEG Studies of Stimulus Evoked Responses
This chapter aims to facilitate the use of MEG for investigating network connectivity in children. Below, we present several examples of MEG studies in children. Each study is concluded with a synopsis regarding the contribution of MEG technology in attaining the aims of the study. It is important to realize that most of the studies employing MEG in pediatric populations are used with a clinical aim and focus on neurological conditions, such as mapping focal or multifocal interictal epilepsy. In this capacity MEG is routinely used for preoperative assessment of somatosensory, auditory, visual and language areas for presurgical mapping. The main goal is to improve postoperative patient outcome (Schwartz et al. 2010). There are numerous MEG reports from clinical studies in epilepsy. These are the focus of another chapter in this volume (see Ivasaki and Nakasato, Chap. 39).
Early pediatric MEG studies focused on sensory processing. For example, Paetau et al. (1995) evaluated the characteristics of the auditory M100 in typically developing children in response to speech and non-speech stimuli. The reported reduction of the M100 latency in children age 3–15 years was suggested to be due to a longer refractory time in the auditory cortex, mostly over the right hemisphere (Rojas et al. 1998). The maturational changes in latency of M100 have also been used to investigate processing of low and high frequency tones in children with Autism Spectrum Disorder (ASD) and in healthy children (Gage et al. 2003a, b). A delayed activation in the auditory cortex in ASD as compared to controls was found. The annual rate of latency reduction with age was slower in the ASD group than in controls, suggesting a continued maturational deficit in autism. Furthermore, we have recently identified a systematic delay in auditory processing in children with fetal alcohol spectrum disorders (Stephen et al. 2012). This finding suggests that sensory processing deficits may be a nonspecific marker of atypical brain development across developmental disorders. The above studies indicate that timing of cortical processing is a critical marker of brain maturational progress and therefore millisecond resolution of MEG measures is invaluable here. In agreement, Roberts (2009) provided evidence for a direct link between white matter development in the auditory white matter tracts and decreases in MEG latency with increasing age. Our results from a younger cohort of children aged 6–50 months provides additional evidence of a linear decrease in latency and evolution of the morphology of auditory evoked responses in very young children (see Fig. 2).
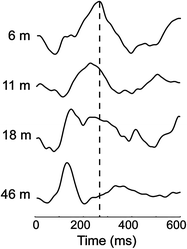
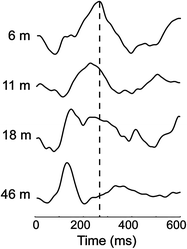
Fig. 2
Auditory evoked responses during sleep in children aged 6–50 months. A linear progression of decreasing latency was observed across 35 healthy children. (Stephen et al. Developmental Neuroscience, submitted)
MEG studies have also characterized the development of the somatosensory response across age. Most notably Pihko et al. (2009), Pihko and Lauronen (2004) described the emergence of the characteristic peaks of the somatosensory response in infants relative to children and adults. Additional studies indicate atypical brain development based on abnormal somatosensory responses in children with autism spectrum disorders (Marco et al. 2012), children with cerebral palsy (Kurz and Wilson 2011), and in children born prematurely (Rahkonen et al. 2013).
Most of the reported pediatric MEG studies focused on cognitive development search for magnetic sources of cortical activity associated with a task. The scalp location of magnetic signals and their coherent changes in distant cortical regions inform researchers about interacting components of cognitive networks. In one study facial stimuli were employed to examine preferences to faces from early childhood (6 y) through young (20–30 y) adulthood (Taylor et al. 2011). Over 200 faces were presented within a 1-back working memory task. The high temporal resolution of MEG permitted careful control of time of onset for cortical components (including face-specific M100 and M170). Contrast between those signals synchronized in time offered an important insight into the location of cortical activity associated with developmental changes in memorizing facial stimuli. The M250–600 ms peak was prominent for repeated faces across all ages and all frequency windows, and larger in the right temporal region including the hippocampus. Thus, well-developed facial recognition is present early in childhood. The engagement of the ventral prefrontal cortex, well documented in memory processing in adults, only became significant in later childhood. Generally consistent with these findings are results from another MEG study pointing to protracted development of face processing until adolescence (Kylliainen et al. 2006). The authors presented stimuli of faces and motorbikes in a visual consecutive matching task in boys 8–11 and young adult men. Although MEG activation in the right ventral occipital-temporal region was engaged in both groups at 100 and 135 ms (M100, M135), the primary evidence for face sensitivity in this region came only from a consistent and narrow timing difference between the responses to faces and motorbikes.
3.2 MEG Studies of Brain Oscillatory Activity in Children
Research by others and by our team indicates that neural oscillations are critical for understanding developmental processes within and between brain networks. Indeed, spectral analysis has long been used to characterize brain development in children mostly using EEG (Case 1992; Klimesch et al. 2001; Krause et al. 2007). A change in amplitude or phase of a single oscillation reflects a change in local neural processing, while amplitude and/or phase correlations (e.g. synchronization) between two distant oscillations reflect the functional connectivity between two neural populations (Buzsaki 2006). An important theta-alpha-beta EEG study in children and adolescents revealed an age-dependent pattern of oscillatory changes (Uhlhaas et al. 2009). These changes involve gradually increasing neural synchrony across childhood followed by an unexpected decrease in synchrony in later adolescence, paralleled by lower performance. The finding is of significance for diagnostic and treatment approaches in child psychopathology, and needs to be further investigated using MEG. Although a considerable number of studies have been completed on oscillatory activity in children using EEG, very few were done with MEG. The general consensus is that the MEG signal is more pure as it is not distorted by skull or skin artifacts, has the advantage of better spatial localization (Papanicolaou et al. 2005a, b) and is superior in analysis of coherence within and between networks (Hamalainen and Hari 2002; Srinivasan et al. 2007). It offers, therefore, unique insight into the mechanism of brain development. For example, our recent MEG study showed a clear increase of mu rhythm frequency in children from 3 months to 5 years of age relative to adults with the most rapid development occurring in the first year of life (Berchicci et al. 2011). This is in line with earlier spectral EEG studies in children (Orekhova et al. 2006; Yordanova and Kolev 1996). Fewer EEG studies have been performed on the sensorimotor mu rhythm, in part because it is only sporadically identified with consistent identification in standard clinical EEG in only 10 % of individuals (Fisch 1991). It has been observed that the generators of the mu rhythm are oriented in the cortex in such a way that MEG is more sensitive than EEG to the sensorimotor mu rhythm. However, further research is needed to explain the developmental significance of this increase in frequency with age and the mu rhythm’s role in the development of imitation skills in young children.
Even more rare are studies investigating developmental changes in spectral oscillatory activity in children performing visual working memory tasks. Among different oscillatory spectra the alpha band is the earliest to develop, most robust in children and displays a clear relationship to the inhibitory brain network (Klimesch et al. 2007; Kolev et al. 2002; Krause et al. 2007; Yordanova et al. 2001; Yordanova and Kolev 1996). Alpha oscillations have been associated in adults with top-down inhibitory control and the frontal–parietal network. We recently examined developmental differences in top-down cognitive control by monitoring MEG event-related desynchronization (ERD) and event-related synchronization (ERS) of alpha-band oscillatory activity (8–13 Hz) during the anticipation stage, target detection stage and post-response stage using a visual working memory task, the Categorical N-Back [CNBT; (Ciesielski et al. 2004, 2006, 2010)]. CNBT was validated in our fMRI studies (Ciesielski et al. 2006), which revealed prime activation in the inferior frontal cortex in adults, but significantly less prominent in children. Children, however, had stronger activation than adults in the striatum and posterior cerebellum (Fig. 3a). For details on CNBT see Sect. 6.4. in this chapter. Full head MEG was recorded from healthy 10-year-old children and young adults, and analyzed with a focus on the frontal-parietal attention network. Figure 3b illustrates the alpha modulation at different temporal stages of the CNBT in children as compared to adults. Whereas adults showed a modulation of the ERD at the anticipatory stages of CNBT and ERS at the post-response stage, children displayed only some anticipatory modulation of ERD but no ERS at the post-response stage, with the alpha-band magnitude remaining in a desynchronized state. Since prior neuroimaging findings indicate that the prefrontal–parietal networks are not fully developed in 10-year olds, and since the children performed as well as the adults on CNBT and yet displayed different patterns of ERD/ERS at different time points of the categorical n-back task (Fig. 3c), it has been suggested that children may be using different top-down cognitive strategies and, hence, different, developmental-stage appropriate neuronal networks (Ciesielski et al., 2006, 2010). One needs to emphasize that top-down cognitive control develops as a result of interaction between top-down and bottom-up networks (Friston and Price 2011; van Essen et al. 1992), and thus new MEG paradigms integrating both measures need to be developed for testing children.
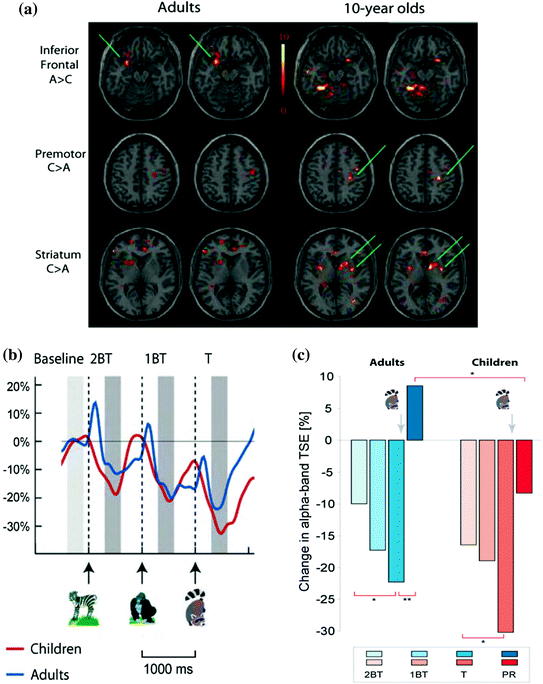
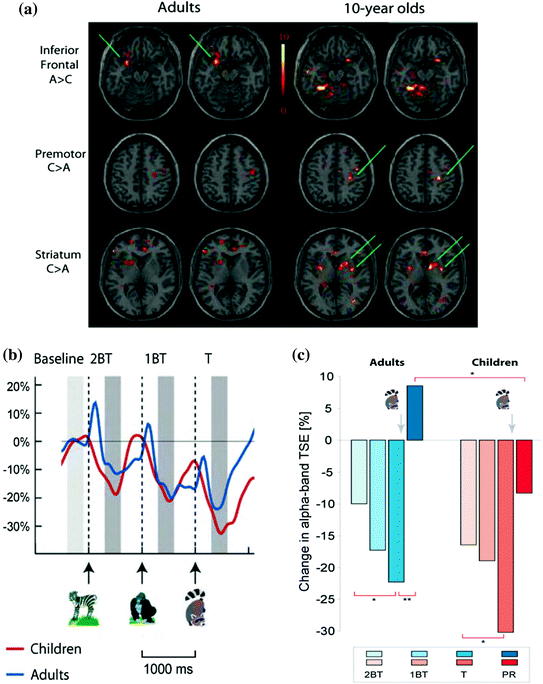
Fig. 3
a CNBT has been validated in fMRI studies with adults (A) and children (C); Of note are the age-dependent differences in task-related networks; b Changes in MEG alpha-band Temporal-Spectral Evolution TSE [%]. The normalized TSE waveforms show task-related changes in alpha-band activity; c Changes in alpha band: BT-before the target, T-Target-raccoon; PR-Post-Response; TSE changes calculated in relation to the Pre-2BT baseline; *p < 0.05; **p < 0.001. Ciesielski et al. (2006, 2010)
Banaschewski and Brandeis (2007) have recently summarized the findings on developmental patterns in oscillatory brain activity noting a decrease in low frequency activity (delta and theta) and an increase in alpha and beta activity with increasing age. Developmental aspects of gamma have been studied less, but are reliably implicated in feature binding (Csibra et al. 2000; Taylor and Baldeweg 2002). Many of the developmental studies have focused on the evolution of sleep patterns throughout development (Danker-Hopfe 2011). Despite differences in spectral patterns across multiple developmental disorders, EEG measures have not reached the threshold to definitively differentiate disorders or to predict long-term outcome (Cantor and Chabot 2009; Rothenberger 2009).
A new focus on spectral analysis in fMRI and the additional temporal resolution afforded by MEG/EEG has led to an increased interest in spectral analysis in EEG and MEG studies to better understand the interaction between resting brain rhythms and cognitive functioning. Recent MEG and EEG studies have also emphasized the importance of accounting for resting brain rhythms in the context of evoked responses (Fujioka and Ross 2008; Kolev et al. 2002). Fujioka and Ross (2008) reported hemispheric differences in alpha desynchronization following pure tones or musical stimuli suggesting that the amount of desynchronization may provide a hemisphere specific measure of brain development. Rojas (2006) also identified an exponential increase in the auditory 40 Hz steady-state response in children providing a potential marker for normal brain development. Recent reviews (Cantor and Chabot 2009; Rothenberger 2009) report evidence that EEG oscillatory measures may play an important role in guiding diagnosis, prevention and treatment of developmental disorders. We postulate that MEG can further facilitate these translational efforts, by better identifying deficits in the spatio-temporal connectome of networks to improve brain functioning in individual children. Future studies will also continue the work linking genetics with cortical oscillatory activity (Begleiter and Porjesz 2006) to identify endophenotypes of developmental disorders.
4 MEG Studies: Challenges Specific to Studies with Children
4.1 MEG: Preparation and Acclimation of Children for Testing: Significance of Parent-Researcher-Child Relationship
MEG has now been used for over a decade to perform clinical diagnostic evaluations in children, but only recently for pediatric research. Excellent communication and trust between the parent, researcher, and child are the prime markers of success in neuroimaging research with children. We, therefore, schedule additional time at the beginning of each MEG session to familiarize both the parent and the child with the study details and testing environment. We describe below several strategies we employ in our pediatric neuroimaging studies to optimize testing for children.
4.2 MEG: Data Acquisition and Processing
Data acquisition is the most important part of any neuroimaging study, since errors committed at this stage are irreversible. Often the participating child is available just for one session. Among the factors that determine whether relevant and valid data have been collected include: clarity of the scientific question and hypothesis, and the pre-selection of a representative subject population. Helpful discussions of those and other factors relevant to studies with children have been published in recent years (Burack et al. 2004; Byars et al. 2002; Cheour et al. 2004; Gaillard et al. 2001; Hansen et al. 2010; Karmiloff-Smith 2010; Kotsoni et al. 2006; Picton et al. 2000; Poldrack 2010; Taylor and Baldeweg 2002; Taylor et al. 2012).
4.3 MEG: Data Acquisition in Infancy
In infants and toddlers [age 6–24 months], an optimal data acquisition is performed during sleep. Multiple studies have confirmed that sensory responses can be probed during sleep with some changes in peak latencies and a reduction in amplitude of later peaks [(Lutter et al. 2004; Pihko et al. 2004)]. Neonatal studies are presented in a separate chapter in this volume (Münbinger et al. in Chap. 23). Importantly, sleep stages mature towards an adult pattern by 6 months of age making it feasible to perform developmental studies across children >6 months while maintaining a constant arousal level across groups and participants. The parent is actively involved and a number of strategies are used for attaining sleep in young children, all aimed at comfort and safety. Infant/child safety must be ensured to reduce the risk of a child rolling off the MEG system beds as only a few are designed with barriers. Some infants may fall asleep better while being held. It is important that the study begins promptly when sensitivity to touch is reduced, as sleep cycles are short in infants (~20 min before they rouse/reposition).
If the MEG study is to be performed while the infant is awake, it is important to schedule the time of day when the infant is most alert with a feeding scheduled immediately prior to the study. Infants are sensitive to diurnal patterns and therefore optimizing the chance for an attentive infant is well worth the effort. Patience of the researchers and parents is a valuable determinant of successful data acquisition. Optimization of the study procedures by eliminating unnecessary steps and having well-trained staff is essential.
4.4 MEG: Data Acquisition in Early Childhood
Young children [ages 3–10 years] are apprehensive of new experiences. Thus, providing the parent, in the presence of a listening child, with comprehensive and friendly study information provides important comfort for a child. To secure child collaboration we designed a specific neuroimaging protocol, for both, MEG and MRI laboratory environments. The protocol reduces movement artifacts and remains enjoyable for the child across the testing sessions. The main highlights of our protocol are: (i) acclimation session conducted a couple of hours prior to MEG or MRI scanning; (ii) relaxation session conducted directly before entering MEG or MRI testing rooms, and (iii) information session with a parent and child about safety and the meaning of MEG and MRI technology. In relation to acclimation, some laboratories use a mock MRI scanner a day or two before testing. In our experience mock MRI may not always work well for young children as upon detecting the difference between the mock and the real scanner, the youngsters may refuse participation. Thus, we conduct the acclimation session in the same MRI scanner the day the study is run. There are no mock MEG helmets, thus exposing the young participant to the actual MEG laboratory environment is the most effective priming technique. Muscle relaxation session is conducted immediately before entering the scanner. Children respond well to instructions of control of targeted groups of muscles. The playful approach to the study effectively substitutes the child’s fear with curiosity, and develops trust to the experimenter. Imaginary play is typical in young children, and the testing session may be turned into a game with the children imagining themselves as astronauts, etc.
The influence of emotional state, and in particular the level of anxiety in a child and his/her parent are powerful confounds during testing. It is our experience that healthy children who evaluate the laboratory visit as less enjoyable generate higher activation in prefrontal brain regions, despite having comparable performance accuracy to children who enjoyed the study. The relaxation session with children leads to reduction of generalized frontal activity (KRC unpublished data from MEG and fMRI studies). We agree with Hinton (2002) about the importance of maintaining high ethical standards in performing pediatric neuroimaging studies. The consent to participate by the parent and child frequently needs individualized explanations.
4.5 Time-Table for MEG Studies with Children Age 5 and Older
To benefit also from the spatial sensitivity of MEG, a range of available source estimation methods can be used to map MEG sensor data to the source-cortex in each child individually, thereby requiring an MRI in addition to the MEG data. The optimal course of events in MEG studies is to schedule both MEG and a short MRI session within proximity of a couple of hours, with an MEG session always conducted first. It is preferable to schedule neuropsychological testing, for the following day. The novelty of the MEG and MRI imaging environment is often taxing to a child and they need to relax before attending to multiple tasks of a neuropsychological battery. Thus, a 2-day schedule appears optimal for a pediatric MEG study: First day: (i) Study consent and assent; (ii) Acclimation sessions for MEG ~10 min and for MRI ~10 min; (iii) Development of child/parent/researcher rapport [including sensory evaluation, demographics, and question/answer session] takes ~30 min; (iv) MEG data acquisition (with preparation) ~40–60 min; (v) Break 30 min; (vi) Anatomical MRI (with preparations) ~30 min; Second day: (i) Psychiatric/clinical evaluation ~1 h; (ii) Resting break ~1 h; and (iii) Neuropsychological testing ~2 h with resting breaks; (iv) Debriefing session with parents and a child.
5 MEG in Children: Technical Considerations
5.1 Helmet Positioning
It is a continuous challenge to optimize signal strength in pediatric studies since the adult MEG systems are not optimized for children. For example, children find it uncomfortable to keep their head back in the helmet and may compromise data quality in the posterior cortex by bringing their head forward. MEG studies in infants are conducted mostly in the supine position. Alternatively, infants 6–18 months of age may sit in an MEG compatible child seat (Imada et al. 2006). We have found that the supine position works well for children up to 5 years of age (Stephen et al. 2012) by allowing the child to better see investigators in the room in addition to the benefit that the head naturally lays in approximately the same position between transient movements due to the concave shape of the helmet. Finally, continuous head position monitoring provides an important advantage for pediatric studies (Stephen et al. 2012; Wehner et al. 2008). By approximately 5 years of age, MEG data may be collected in the upright position, in agreement with the child’s preference.
5.2 Head Position Indicator Coils
Use of head position indicator (HPI) coils is important to determine where the head is relative to the sensor array in all MEG studies. However, placement of the HPI coils is a greater challenge in children. HPI coils placed on the forehead or neck in young children may be located below the sensor array making the HPI coil position unreadable during data collection. Therefore, we place the HPI coils on the EEG cap, in the case of simultaneous MEG and EEG data collection, or HPI coils are taped to a snug fitting cap that is secured to the head. The cap must be sufficiently taut to ensure that the relative position of the HPI coils does not change with child movement. Beginning from age 5 a child can comfortably sit in the MEG with the HPI coils generally taped along the hairline.
5.3 Bipolar EEG Channels
Bipolar EEG electrodes are used to monitor eye movement, cardiac signal and chin movement in young infants to monitor sleep stage (Fisch 1991). Additional bipolar EEG channels can be used to monitor muscle activity. Based on the robust eye movements and cardiac signals, only mild removal of skin oils is sufficient in infants and young children. We use medical paper tape for attaching facial electrodes so that it can be removed from the skin without distress. Baby oil or commercially-available tape removal swabs provide the best means for removing tape. For older children standard electrode placement is sufficient. However, in childhood disorders with sensory sensitivities electrode placement should be minimized.
6 Examples of Tasks Useful in Pediatric MEG Studies
6.1 Design of Passive Paradigms for Infants
Since infants cannot follow explicit instructions, MEG protocols for infants need to encourage natural behaviors or employ a passive design. Passive designs have been used to track sensory development during sleep in young neonates (see chapter on Designing MEG Experiments by J. Stephen in Chap. 5). Other work has been performed in older infants in the awake state [e.g. (Imada et al. 2006; Johnson et al. 2010, 2013; Wakai et al. 2007)]. Our approach is to track the child’s behavior during the task using: (i) MEG-synchronized video recording to allow for post-processing of behavior with respect to task stimuli and brain function, and (ii) Physiological monitoring, such as monitoring respiration, heartbeat, and supplementary bipolar EEG electrode placements. Standard sleep electrodes are recommended for sleep stage characterization in children who sleep during the protocol. Protocols for young children can include explicit tasks, but require succinct tasks that are designed with young children in mind.
6.2 Sensory Tasks
With some modifications of adult protocols, standard sensory tasks can be presented very efficiently when a child is alert and cooperative. Since cortical processing in children is slower than adults, the inter stimulus interval must be longer to obtain the full sensory response (including the later components). For example, Pihko et al. (2004) recommends an ISI ~2 s for young children. One approach to record data for longer periods of time employed in both child and adult studies is to present a silent video [children: (Oram Cardy et al. 2008; Stephen et al. 2012); adults: (Huttunen et al. 1999; Korvenoja et al. 1999)]. The silent movie while passively activating the visual system is not synchronized to auditory or somatosensory stimuli. It is now recognized that attention impacts even basic sensory responses (Donohue et al. 2011), therefore attention components may be modulated by observation of a video. Our experience is that videos help to calm children and allow for 15 min of data collection in children as young at 2–3 years of age.
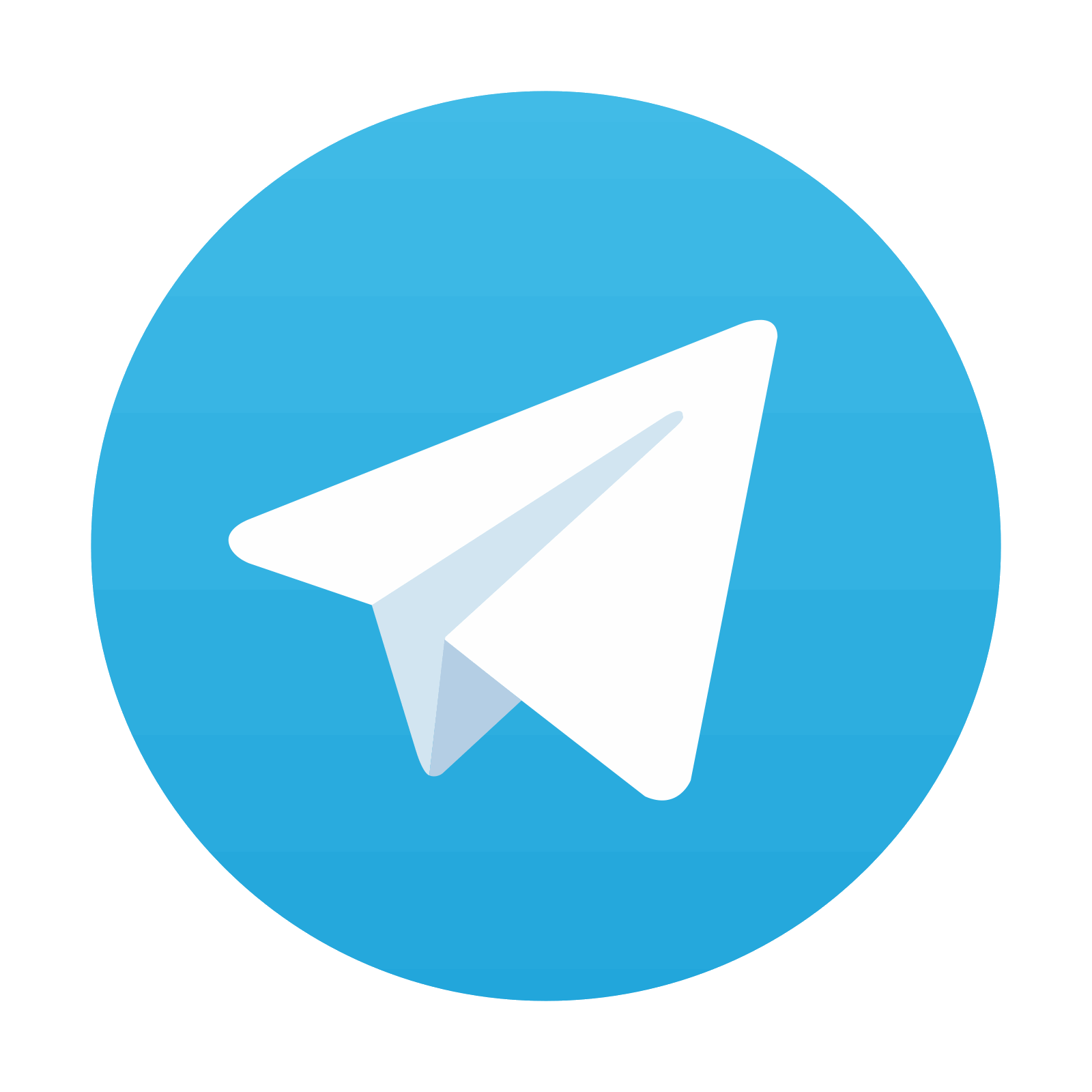
Stay updated, free articles. Join our Telegram channel
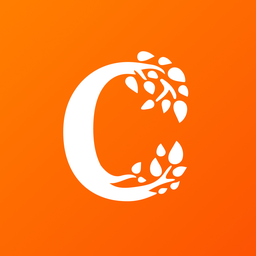
Full access? Get Clinical Tree
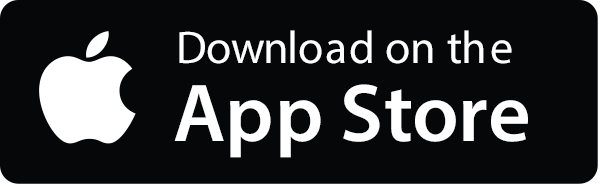
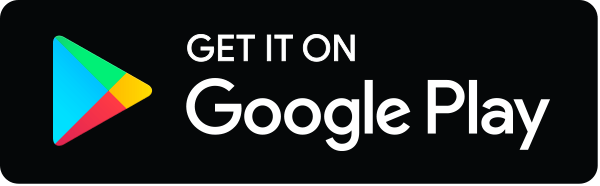