The authors discuss eight areas of quantitative MR imaging that are currently used (RECIST, DCE-MR imaging, DSC-MR imaging, diffusion MR imaging) in clinical trials or emerging (CEST, elastography, hyperpolarized MR imaging, multiparameter MR imaging) as promising techniques in diagnosing cancer and assessing or predicting response of cancer to therapy. Illustrative applications of the techniques in the clinical setting are summarized before describing the current limitations of the methods.
Key points
- •
The fundamental limitations of RECIST (Response Evaluation Criteria in Solid Tumors) must be addressed by more quantitative imaging methods; MR imaging offers many existing and emerging methods to fill this need.
- •
DCE-MR imaging, DSC-MR imaging, and diffusion MR imaging have advanced to the point where they can offer quantitative insights into tumor characteristics, and these techniques are now frequently used in clinical trials either alone or in concert.
- •
Magnetic resonance (MR) elastography, CEST (chemical exchange saturation transfer), and hyperpolarized MR imaging are 3 emerging techniques that can offer insights complementary to those provided by the diffusion and perfusion MR imaging methods.
- •
The ability to acquire multiple data types in a single MR imaging session provides the opportunity to combine these methods in a multiparametric approach, which has been shown to have increased clinical value over single parameter methods.
Incorporating quantitative MR imaging in clinical trials
The last decade has seen tremendous interest and research effort devoted to the use of quantitative imaging within oncology. Quantitative imaging techniques can measure various properties within medical images that might serve as reliable surrogates for various pathophysiological processes with which to personalize cancer therapy and accelerate drug development. Furthermore, the prospect of combining several quantitative imaging measures for establishing radiologic phenotypes predictive of clinical trajectories is particularly appealing, and MR imaging has emerged as a promising modality for this purpose. MR imaging continues to be a mainstay for conventional size-based tumor assessments (ie, the Response Evaluation Criteria in Solid Tumors, RECIST; see next section) that are standard efficacy endpoints within clinical trials and increasingly common in routine, standard-of-care settings. Moreover, MR imaging applications that can quantitatively report on various aspects of tumor biology, including perfusion, cellularity, metabolism, and protein deposition, offer the potential to supplement and enhance conventional anatomic information, which when used alone provides an incomplete assessment of solid tumors. This review spotlights some of the leading technological developments in MR imaging that are laying the groundwork for quantitative MR imaging to transition from being viewed as an advanced research paradigm to becoming a widely established clinical reality for the cancer community. This transition presents a number of unique challenges as well as exciting opportunities for imaging science.
There are several key steps for the development and evaluation of a particular quantitative imaging measure before it can be considered a true biomarker and safely incorporated into clinical practice ( Box 1 ). Perhaps one of the most essential tools for the evaluation of biomarkers is the multicenter clinical trial. Over the last several years, the National Cancer Institute (NCI) has spearheaded efforts to coordinate multicenter biomarker studies for imaging. The NCI’s Quantitative Imaging Network currently includes 17 Centers of Imaging Excellence in the United States, several of which are actively engaged in validation of imaging based biomarkers. Other groups, including the Radiological Society of North America Quantitative Imaging Biomarker Alliance and the American College of Radiology Imaging Network, recently merged with the Eastern Cooperative Oncology Group to form ECOG-ACRIN, have also been rigorously pursuing multicenter quantitative imaging clinical trials. These groups have developed their own clinical trial designs and workflows, image acquisition and analysis procedures, and regulatory processes. There are also efforts to harmonize procedures and practices across these groups in order to arrive at a comprehensive set of standards for the clinical validation and implementation of quantitative imaging biomarkers. The authors draw from collective insights to emphasize a few key commonalities with their own experiences and discuss some administrative, regulatory, and logistical considerations facing trials of a putative MR imaging biomarker.
- 1.
Validation tests the accuracy, precision, repeatability, and reproducibility of the biomarker measurement.
- 2.
Qualification establishes the biomarker as a surrogate for tumor pathophysiology, response to therapy, or other clinical endpoint of interest.
- 3.
Utilization examines the performance and implementation of the biomarker within the specific context of its proposed use, especially across multiple institutions and clinical settings.
One of the most crucial aspects of successful integration of MR imaging biomarker research with an oncology clinical trial will be the level of engagement and collaboration the imaging scientists and radiologists have with the medical oncologists and clinical trial sponsors. Investigator-initiated trials offer certain advantages in this regard relative to industry-sponsored trials, because the latter often requires a higher level of engagement and a greater emphasis on allocating resources for data management and regulatory compliance. Furthermore, because these studies are frequently designed at the industrial sponsor months—or years—before academic investigators become aware of it, integrating an advanced imaging technique can be difficult. Regardless of the type of trial, there are several characteristics pertaining to the design and execution of an MR-based imaging biomarker study that need to be considered within the context of a therapeutic oncology trial. Ideally, the biomarker study design would be rationally tailored to address a clearly defined clinical problem (eg, predicting which patients will benefit from neoadjuvant therapy) and would test the ability of a candidate MR imaging technique, or group of techniques, to predict or correlate with a desired clinical outcome (eg, pathologic complete response). The predictive value of a particular imaging measure will likely vary depending on the choice of clinical endpoint. When progression-free or overall survival (OS) is a primary endpoint, incorporation of multiple strategically chosen imaging time points during follow-up is recommended ( Box 2 ).
- 1.
The expected mechanism, onset, and duration of action of the therapeutic agent or intervention under investigation in the clinical trial.
- 2.
The schedule of events (ie, timing of biomarker scan should coincide whenever possible with the patient’s clinical appointments) within the trial.
- 3.
Potential interference from use of contrast media in other clinical trial radiological procedures.
- 4.
Interscan interval in relation to reimbursement policies of the sponsor, patient’s insurance provider, or Centers for Medicate and Medicaid Services.
The first scientific body to review, advise, and ultimately approve an imaging-based biomarker study is the institutions’ Scientific Review Committee (SRC), which consists of clinicians, basic scientists, biostatisticians, nurses, pharmacists, and other medical professionals whose primary mission is to scrutinize the scientific merit and clinical prioritization of a new study in the context of an institution’s existing menu of studies. For most institutions, a new clinical trial protocol will undergo review by the SRC before it is reviewed by the local Institutional Review Board (IRB). A critical aspect of the SRC review entails thorough scrutiny of the statistical methodology proposed in the study. Before protocol submission, it is highly worthwhile, and at some institutions required, to meet with a qualified biostatistician, particularly one well versed in the analysis of imaging data, to ensure that the aims and study design are in keeping with a sound statistical framework appropriate for the development of imaging biomarkers. Studies must be demonstrated as having accrual goals capable of satisfying a predefined level of statistical power (typically, 80%) to determine the predictive association between the MR biomarker or biomarkers in question and the primary clinical endpoint. Sample sizes must also be justified on the basis of historical accrual data within identical or very similar patient populations and clinical settings. Feedback from the SRC is one of the primary opportunities for constructive criticism so that the aims, design, and future conduct of a study are consistent with institutional standards for statistical rigor, clinical relevance, and scientific quality.
Once SRC approval is obtained, the next step in opening a new imaging study is protocol review and approval by the local IRB. Although scientific rigor and clinical relevance are the primary concerns in SRC review, the IRB is typically most focused on ensuring that the study meets all federal, state, and local policies pertaining to patient safety and confidentiality. For MR imaging studies, one of the most important aspects of IRB review will be centered on the patient screening process to ensure compatibility with the large magnetic field the patient will encounter as part of the imaging procedure. The IRB will verify that prospective patients will be given every means necessary to disclose the presence of ferromagnetic materials and will also allow designated key study personnel to access the prospective patient’s medical record to verify MR compatibility of implants; this is especially important in patients with cancer receiving chemotherapy or other procedures (biopsy, surgery), because vascular access ports, biopsy marker clips, and stents are constructed of materials whose MR compatibility can vary widely. The IRB will heavily scrutinize methods for verifying the manufacturer and model number for an implant or device in question. Rigorous rating standards from the American Society for Testing and Materials (ASTM) International exist for virtually all implantable biomaterials and have been approved by the US Food and Drug Administration. The IRB will mandate that only implanted materials having an ASTM rating of “MR Safe” at the particular field strength in question are included within the study. Implants having a rating of “MR Conditional” are often excluded, but there are instances where a particular MR imaging environment with specific conditions may be acceptable. For example, at 3.0 T, the only implants or devices currently accepted on study are those classified as MR Conditional 6 (ASTM Standard F2503).
While the IRB is reviewing the imaging protocol, there are several steps that can be taken to help ensure no delays are experienced in opening to accrual. Like therapeutic trials, studies devoted to validating a perspective imaging biomarker should be nationally registered at ClinicalTrials.gov and/or the NCI. At many institutions, national study registration is required of all clinical studies as a matter of local policy, and recent changes to the registration rules put forward by the International Committee of Medical Journal Editors now necessitate registration even for noninterventional imaging studies. Another critical logistical step before opening to accrual is to ensure that all imaging-related study materials have been distributed to the appropriate study personnel. The most convenient venue for such interactions is the Site Initiation Visit, where imaging scientists, clinicians, research nurses, and others can meet to review key aspects of the study to establish an adequate recruitment plan and clinical workflow. This Site Initiation Visit is particularly important in situations whereby the acquisition of imaging data is to occur on dedicated research scanners in facilities that are separated from the cancer clinics where recruitment will take place, because the processes and procedures involved in advanced imaging studies are often unfamiliar to clinical staff.
Incorporating quantitative MR imaging in clinical trials
The last decade has seen tremendous interest and research effort devoted to the use of quantitative imaging within oncology. Quantitative imaging techniques can measure various properties within medical images that might serve as reliable surrogates for various pathophysiological processes with which to personalize cancer therapy and accelerate drug development. Furthermore, the prospect of combining several quantitative imaging measures for establishing radiologic phenotypes predictive of clinical trajectories is particularly appealing, and MR imaging has emerged as a promising modality for this purpose. MR imaging continues to be a mainstay for conventional size-based tumor assessments (ie, the Response Evaluation Criteria in Solid Tumors, RECIST; see next section) that are standard efficacy endpoints within clinical trials and increasingly common in routine, standard-of-care settings. Moreover, MR imaging applications that can quantitatively report on various aspects of tumor biology, including perfusion, cellularity, metabolism, and protein deposition, offer the potential to supplement and enhance conventional anatomic information, which when used alone provides an incomplete assessment of solid tumors. This review spotlights some of the leading technological developments in MR imaging that are laying the groundwork for quantitative MR imaging to transition from being viewed as an advanced research paradigm to becoming a widely established clinical reality for the cancer community. This transition presents a number of unique challenges as well as exciting opportunities for imaging science.
There are several key steps for the development and evaluation of a particular quantitative imaging measure before it can be considered a true biomarker and safely incorporated into clinical practice ( Box 1 ). Perhaps one of the most essential tools for the evaluation of biomarkers is the multicenter clinical trial. Over the last several years, the National Cancer Institute (NCI) has spearheaded efforts to coordinate multicenter biomarker studies for imaging. The NCI’s Quantitative Imaging Network currently includes 17 Centers of Imaging Excellence in the United States, several of which are actively engaged in validation of imaging based biomarkers. Other groups, including the Radiological Society of North America Quantitative Imaging Biomarker Alliance and the American College of Radiology Imaging Network, recently merged with the Eastern Cooperative Oncology Group to form ECOG-ACRIN, have also been rigorously pursuing multicenter quantitative imaging clinical trials. These groups have developed their own clinical trial designs and workflows, image acquisition and analysis procedures, and regulatory processes. There are also efforts to harmonize procedures and practices across these groups in order to arrive at a comprehensive set of standards for the clinical validation and implementation of quantitative imaging biomarkers. The authors draw from collective insights to emphasize a few key commonalities with their own experiences and discuss some administrative, regulatory, and logistical considerations facing trials of a putative MR imaging biomarker.
- 1.
Validation tests the accuracy, precision, repeatability, and reproducibility of the biomarker measurement.
- 2.
Qualification establishes the biomarker as a surrogate for tumor pathophysiology, response to therapy, or other clinical endpoint of interest.
- 3.
Utilization examines the performance and implementation of the biomarker within the specific context of its proposed use, especially across multiple institutions and clinical settings.
One of the most crucial aspects of successful integration of MR imaging biomarker research with an oncology clinical trial will be the level of engagement and collaboration the imaging scientists and radiologists have with the medical oncologists and clinical trial sponsors. Investigator-initiated trials offer certain advantages in this regard relative to industry-sponsored trials, because the latter often requires a higher level of engagement and a greater emphasis on allocating resources for data management and regulatory compliance. Furthermore, because these studies are frequently designed at the industrial sponsor months—or years—before academic investigators become aware of it, integrating an advanced imaging technique can be difficult. Regardless of the type of trial, there are several characteristics pertaining to the design and execution of an MR-based imaging biomarker study that need to be considered within the context of a therapeutic oncology trial. Ideally, the biomarker study design would be rationally tailored to address a clearly defined clinical problem (eg, predicting which patients will benefit from neoadjuvant therapy) and would test the ability of a candidate MR imaging technique, or group of techniques, to predict or correlate with a desired clinical outcome (eg, pathologic complete response). The predictive value of a particular imaging measure will likely vary depending on the choice of clinical endpoint. When progression-free or overall survival (OS) is a primary endpoint, incorporation of multiple strategically chosen imaging time points during follow-up is recommended ( Box 2 ).
- 1.
The expected mechanism, onset, and duration of action of the therapeutic agent or intervention under investigation in the clinical trial.
- 2.
The schedule of events (ie, timing of biomarker scan should coincide whenever possible with the patient’s clinical appointments) within the trial.
- 3.
Potential interference from use of contrast media in other clinical trial radiological procedures.
- 4.
Interscan interval in relation to reimbursement policies of the sponsor, patient’s insurance provider, or Centers for Medicate and Medicaid Services.
The first scientific body to review, advise, and ultimately approve an imaging-based biomarker study is the institutions’ Scientific Review Committee (SRC), which consists of clinicians, basic scientists, biostatisticians, nurses, pharmacists, and other medical professionals whose primary mission is to scrutinize the scientific merit and clinical prioritization of a new study in the context of an institution’s existing menu of studies. For most institutions, a new clinical trial protocol will undergo review by the SRC before it is reviewed by the local Institutional Review Board (IRB). A critical aspect of the SRC review entails thorough scrutiny of the statistical methodology proposed in the study. Before protocol submission, it is highly worthwhile, and at some institutions required, to meet with a qualified biostatistician, particularly one well versed in the analysis of imaging data, to ensure that the aims and study design are in keeping with a sound statistical framework appropriate for the development of imaging biomarkers. Studies must be demonstrated as having accrual goals capable of satisfying a predefined level of statistical power (typically, 80%) to determine the predictive association between the MR biomarker or biomarkers in question and the primary clinical endpoint. Sample sizes must also be justified on the basis of historical accrual data within identical or very similar patient populations and clinical settings. Feedback from the SRC is one of the primary opportunities for constructive criticism so that the aims, design, and future conduct of a study are consistent with institutional standards for statistical rigor, clinical relevance, and scientific quality.
Once SRC approval is obtained, the next step in opening a new imaging study is protocol review and approval by the local IRB. Although scientific rigor and clinical relevance are the primary concerns in SRC review, the IRB is typically most focused on ensuring that the study meets all federal, state, and local policies pertaining to patient safety and confidentiality. For MR imaging studies, one of the most important aspects of IRB review will be centered on the patient screening process to ensure compatibility with the large magnetic field the patient will encounter as part of the imaging procedure. The IRB will verify that prospective patients will be given every means necessary to disclose the presence of ferromagnetic materials and will also allow designated key study personnel to access the prospective patient’s medical record to verify MR compatibility of implants; this is especially important in patients with cancer receiving chemotherapy or other procedures (biopsy, surgery), because vascular access ports, biopsy marker clips, and stents are constructed of materials whose MR compatibility can vary widely. The IRB will heavily scrutinize methods for verifying the manufacturer and model number for an implant or device in question. Rigorous rating standards from the American Society for Testing and Materials (ASTM) International exist for virtually all implantable biomaterials and have been approved by the US Food and Drug Administration. The IRB will mandate that only implanted materials having an ASTM rating of “MR Safe” at the particular field strength in question are included within the study. Implants having a rating of “MR Conditional” are often excluded, but there are instances where a particular MR imaging environment with specific conditions may be acceptable. For example, at 3.0 T, the only implants or devices currently accepted on study are those classified as MR Conditional 6 (ASTM Standard F2503).
While the IRB is reviewing the imaging protocol, there are several steps that can be taken to help ensure no delays are experienced in opening to accrual. Like therapeutic trials, studies devoted to validating a perspective imaging biomarker should be nationally registered at ClinicalTrials.gov and/or the NCI. At many institutions, national study registration is required of all clinical studies as a matter of local policy, and recent changes to the registration rules put forward by the International Committee of Medical Journal Editors now necessitate registration even for noninterventional imaging studies. Another critical logistical step before opening to accrual is to ensure that all imaging-related study materials have been distributed to the appropriate study personnel. The most convenient venue for such interactions is the Site Initiation Visit, where imaging scientists, clinicians, research nurses, and others can meet to review key aspects of the study to establish an adequate recruitment plan and clinical workflow. This Site Initiation Visit is particularly important in situations whereby the acquisition of imaging data is to occur on dedicated research scanners in facilities that are separated from the cancer clinics where recruitment will take place, because the processes and procedures involved in advanced imaging studies are often unfamiliar to clinical staff.
Current use of MR imaging for clinical trials
In modern clinical oncology practice, MR imaging is widely used as a tool for cancer screening, lesion detection, lesion characterization, and therapy monitoring. Within cancer clinical trials, MR imaging is used for assessing response to treatment, although its role varies depending on the anatomic site of disease. For many clinical trials in solid malignancies, MR imaging may play a secondary role to computed tomography (CT) and may be used only when there is a contraindication to iodinated intravenous contrast media. For certain tumor types (eg, brain and head/neck cancers), MR imaging may be the preferred modality for response assessment due to its excellent soft tissue contrast resolution.
When MR imaging is used for treatment response assessment, most current cancer clinical trials use one of several standardized response assessment guidelines based on changes in gross lesion size. These guidelines specify how to identify and measure target lesions at baseline imaging before therapy, how to evaluate disease burden at follow-up time points following initiation of treatment, and how to place patients into response categories at successive time points over the course of the clinical trial. The most widely used response assessment guideline, incorporated into most modern solid tumor clinical trials, is RECIST. Because its emphasis is on changes in tumor size measurement over time, RECIST necessitates high-spatial resolution MR imaging techniques optimized for capturing anatomic detail.
Measuring changes in tumor size on anatomic imaging has been the mainstay of imaging-based response assessment for decades and is supported by research linking tumor shrinkage in early-stage trials with subsequent survival benefits. However, an exclusive focus on anatomic imaging has recently been called into question with the emergence of functional imaging techniques that provide information on tumor status beyond lesion size. These techniques, many of which are MR-based and are described later, offer the promise of reporting on response at an earlier time point than traditional tumor size-based approaches, which may lag weeks to months behind a physiologic tumor response. Functional imaging techniques may also succeed in better capturing and measuring the antitumor efficacy of newer targeted agents, the cytostatic effects of which may be underestimated by traditional size-based approaches.
Quantitative MR imaging techniques currently available for clinical trials
Three techniques are focused on that have advanced to the point where they are frequently used in clinical trials to report on therapeutic response. In the section entitled “Emerging MR Imaging Methods for Cancer,” 4 emerging techniques are described.
Dynamic Susceptibility Contrast MR Imaging
Abnormal angiogenesis is a common characteristic of malignant brain tumors, and dynamic susceptibility contrast MR imaging (DSC-MR imaging) is frequently used to noninvasively interrogate the hemodynamic features of the expanding vascular network. In DSC-MR imaging, dynamic MR images are acquired before and after an intravenous bolus injection of a contrast agent (CA), which is typically one of several clinically approved gadolinium chelates. As the CA passes through tissue, it decreases the relaxation times (T 1 , T 2 , and T 2 ∗ ) of tissue water and the associated MR imaging signal intensity. The magnitude of the change in the relaxation rate is determined by the concentration of the CA and the geometry of the tissue structures containing the CA. Pharmacokinetic models can be applied to DSC-MR imaging data to estimate blood volume, blood flow, and mean transit time.
Given the known association between brain tumor pathologic abnormality and angiogenesis, early DSC-MR imaging studies demonstrated the clinical utility of this technique by verifying a positive correlation between tumor blood volume and brain tumor grade. As an example, Boxerman and colleagues found relative blood volume values (ie, relative to normal appearing white matter) of 1.52, 2.84, and 3.96 in a cohort of patients with World Health Organization grades II (n = 11), III (n = 9), and IV (n = 23), respectively. Furthermore, the correlation between blood volume and tumor grade was significant (r = 0.60; P <.0001). It was noted that designating tumor grade based on blood volume maps alone, however, may be confounded by intragrade variability, particularly between grades III and IV.
Although such diagnostic studies served to support the consideration of DSC-MR imaging in brain tumor patient management, its clinical potential was more fully realized when studies emerged demonstrating its prognostic capabilities. Law and colleagues investigated the ability of pretreatment blood volume maps to predict clinical response (complete response, stable disease, progressive disease [PD], and death) in patients with low-grade gliomas undergoing standard-of-care treatments. The patients with lesions exhibiting blood volume values less than 1.75 (relative to normal appearing white matter) had a median time to progression of 4620 days, whereas those with values higher than 1.75 had a median time to progression of 245 days. An important conclusion in this study is that while DSC-MR imaging may have low specificity for diagnosing low-grade gliomas, it has a much higher specificity for predicting clinical endpoints in patients receiving standard treatment regimens.
In the context of routine therapy and clinical trials, standard MR imaging techniques are unable to reliably differentiate between PD and pseudoprogression (PsP). Because of the heightened angiogenic response in recurring gliomas, DSC-MR imaging–derived cerebral blood volume (CBV) maps have been explored as a means to overcome this limitation. In a phase II clinical trial of temozolomide, paclitaxel poliglumex, and concurrent radiation, the mean CBV measured at initial progressive enhancement and the change in CBV after therapy were used to distinguish PD and PsP. The single time point CBV values acquired after therapy were similar between patients exhibiting PsP and PD (2.35 vs 2.17, P = .67). However, changes in CBV between follow-up examinations were significantly different between PsS and PD (−0.84 and 0.84, P = .001) as were the trends in CBV (negative vs positive slope; P = .04). It was concluded that longitudinal changes in posttherapy CBV values may be more useful for tracking treatment response than static values ( Fig. 1 ).
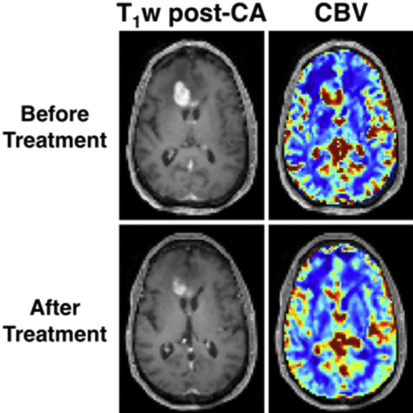
The identification of early predictors of clinical endpoints (eg, OS) could reduce the duration and cost of clinical trials. Toward this end, the predictive potential of DSC-MR imaging in glioblastoma multiforme (GBM) patients was recently evaluated in ACRIN 6677/RTOG 0625, a multicenter, randomized, phase II trial of bevacizumab with irinotecan or temozolomide. Changes in tumor CBV before and at 2, 8 and 16 weeks after treatment initiation were correlated with OS. Significant decreases in CBV at 2 weeks were observed in patients with an OS greater than 1 year, whereas patients with increases in tumor CBV were found to have significantly shorter OS. This trial highlights the potential of CBV as a prognostic biomarker of treatment response in recurrent GBM patients, particularly in the context of therapeutic agents targeting angiogenic pathways.
With the increasing use of DSC-MR imaging in clinical trials and routine practice, there is growing interest in the field to standardize image acquisition and postprocessing strategies. Although there is a general consensus on the most robust acquisition strategies (eg, pulse sequence type and parameters, CBV quantification, correction techniques for CA leakage effects), current efforts aim to address the challenges of harmonizing these techniques across MR imaging vendors and data analysis packages.
Dynamic Contrast-Enhanced MR Imaging
DCE-MR imaging acquires heavily T 1 -weighted images before, during, and after injection of a CA leading to an increase in signal intensity on T 1 -weighted images yielding a time-intensity curve reflecting the delivery and retention of CA within the tissue of interest. DCE-MR imaging is a class of techniques characterized by whether a qualitative, semiquantitative, or quantitative approach is used for data analysis. A qualitative analysis examines the shape (eg, plateau or persistent) of the time-intensity curve, while a semi-quantitative analysis provides values such as the area under the curve (AUC), enhancement, time to peak, and wash-in/wash-out slopes. A quantitative analysis fits the time-intensity curve to pharmacokinetic models to extract parameters that reflect physiologic characteristics, such as tumor vessel perfusion and permeability and tissue volume fractions. Although applying quantitative models to the DCE-MR imaging data is more complex than qualitative or semiquantitative approaches, the extracted parameters provide (in principle) a more direct measure of vascular characteristics. The Tofts-Kety model is most frequently used and considers the CA distributed between 2 compartments, the blood/plasma space (C p ) and the tissue space (C t ). In 1999, Tofts and colleagues standardized quantitative DCE-MR imaging notation where K trans is the volume transfer constant between C p and C t , k ep is the redistribution rate constant between C t and C p , and the plasma and tissue volume fractions are denoted as v p and v e , respectively.
DCE-MR imaging has played a role in the assessment of anticancer therapies as well as in the prediction of eventual response in a variety of cancers ( Fig. 2 ). An early report using DCE-MR imaging as a study endpoint in a phase I clinical trial was in 2002 when 5,6-dimethylxanthenone-4-acetic acid was used to treat patients with advanced solid tumors. Despite the small sample size, significant reductions in the AUC were reported in 9 of the 16 patients at 24 hours after the first dose. More recently, DCE-MR imaging was investigated in a phase I trial of patients with prostate cancer treated with cediranib. In most of the patients, Dahut and colleagues observed rapid and sustained reductions in AUC and K trans from baseline up to 2 or more cycles of therapy. In addition, K trans at baseline was associated with progression-free survival, suggesting that DCE-MR imaging may also be a predictive biomarker of clinical outcome. DCE-MR imaging was found to be predictive of pathologic complete response (pCR) in patients with stage II/III breast cancer undergoing neoadjuvant chemotherapy. Li and colleagues found that after one cycle of therapy, k ep predicted pCR with a sensitivity and specificity of 0.83 and 0.65, respectively.
Even with these successes, several limitations of DCE-MR imaging have been identified emphasizing the need for its systematic evaluation in assessing treatment response and predicting clinical outcomes. Accuracy and precision of the estimated quantitative parameters can be affected by the estimation of the arterial input function, spatial and temporal resolutions, pharmacokinetic models, and curve fitting strategies. On the subject of model fitting, Huang and colleagues were the first to compare 12 DCE-MR imaging software tools in a multicenter data analysis challenge. K trans , k ep , v e , and v p from 10 patients before and after the first cycle of neoadjuvant chemotherapy (BAC) were analyzed using site-specific models and algorithms. Although considerable parameter variations were observed, agreement in parameter percentage change was better than that in absolute parameters. Further systematic evaluations assessing reproducibility, evaluating efficacy in a specific patient population and therapy, and finally, expanding into a multicenter study are required. Reproducibility studies are important in order to establish the range outside of which any observed changes would be due to therapy and not measurement error. The reproducibility of several semiquantitative and quantitative parameters has been investigated in patients with solid tumors. Although there have been some excellent efforts at evaluating semiquantitative DCE-MR imaging in a large multicenter trial (see Ref. ), more studies are needed before DCE-MR imaging can be fully used in routine clinical care.
Diffusion-Weighted Imaging
In diffusion-weighted imaging (DWI), the image contrast reflects the distance water molecules can migrate or diffuse from their original spatial position over a short time interval due to random, thermally induced motion (ie, Brownian motion). By acquiring 2 or more images with different degrees of diffusion weighting (obtained by applying the diffusion sensitizing gradients with different amplitudes on successive image acquisitions), an estimate of the amount of molecular water diffusion, termed the apparent diffusion coefficient (ADC), can be calculated at each voxel using the equation
where S is the signal intensity measured with application of a diffusion-sensitizing gradient, S 0 is the signal intensity with no diffusion-sensitizing gradient, and b is a composite variable reflecting various acquisition parameters (including the strength of the gradient pulse, duration of the pulse, and interval between pulses). For a more extensive review of the physics of DWI, the reader is referred to Ref.
Cancers often exhibit significantly reduced ADC values when compared with healthy tissues, a finding typically attributed to the increased cell density of many malignancies. With treatment, intratumoral ADC values typically increase, presumably because of decreases in cell density consequent to apoptosis and cell death, with concomitant disruption of cell membranes, allowing water molecules to diffuse more freely. This basic paradigm—low tumor ADC values before treatment followed by rising tumor ADC values with effective treatment—provides the basic model for DWI as a technique for response assessment ( Fig. 3 for an illustrative example).
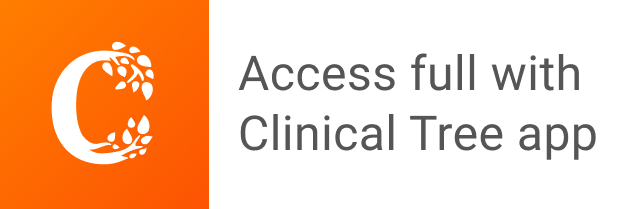