Fig. 5.1
Schematic overview of MRI and PET imaging potential
5.1 MRI Basics
5.1.1 Nuclear Magnetic Resonance (NMR) (Gibby 2005; Pooley 2005.; McRobbie 2003; NessAiver 1997; Elster and Burdette 2001)
Nuclei with an odd mass or odd atomic number have an intrinsic spin angular moment with an associated magnetic moment. In the presence of an external magnetic field B0, the magnetic moment of nuclei with a one half spin will line up in parallel with the applied magnetic field with an either spin-aligned or spin-opposed orientation. The energy difference between the two orientations is very small at room temperature with a small majority of nuclei populating the lower energy spin-aligned orientation. This will cause a net macroscopic magnetization M0 which will be directed parallel to B0 and which strength will depend on the spatial spin density and the magnetic field strength. Next to this magnetization, nuclei will tend to precess in phase around the magnetic field with a frequency depending on the nucleus, the molecular structure, and the strength of the magnetic field. This frequency is traditionally called the Larmor frequency. Generating a MR signal is a two-phase process. During the transmission phase, a radiofrequency (RF) signal induces a “spin flip” resulting in a rotating transversal magnetization in the plane perpendicular to the longitudinal external magnetic field B0 and in a residual longitudinal component if the flip angle (FA) is not 90°. During the receiving phase, the transversal magnetization is picked up to generate the MR signal. During transmission, the RF excitation frequency must fit the Larmor frequency to have optimal resonance conditions, hence the term magnetic resonance. The Larmor frequency for the 1H nucleus amounts to 42.58 MHz/Tesla.
After excitation and during the receiving phase, relaxation occurs when magnetization returns to the state prior to RF excitation. One can differentiate two types of relaxation. Longitudinal relaxation describes the relaxation where the longitudinal magnetization component is exponentially restored to initial net longitudinal magnetization M0. This relaxation is referred to as spin-lattice relaxation and is quantified by the longitudinal relaxation time constant T1. Transverse relaxation corresponds to the so-called spin-spin relaxation and describes the exponential decrease of the transverse magnetization component perpendicular to the longitudinal external magnetic field. The representative parameter is the transverse relaxation time constant T2. Both relaxation processes take place simultaneously with relaxation times T1 and T2 being characteristic for a specific tissue type. While the T1 relaxation time is within the range of 300 ms (fat) to 3,000 ms (fluids), T2-relaxation times are always much shorter ranging from 30 ms (muscle) to 2,000 ms (fluids) (Bazelaire et al. 2004). Specifically for brain imaging and for a 3 T magnetic field, T1 relaxation times are 832 msec for white matter and 1,331 ms for gray matter, while T2-relaxation times are 79.6 and 110 ms, respectively (Wansapura et al. 1999).
5.1.2 Magnetic Resonance Imaging (MRI) (Paschal and Morris 2004; Hennig 1999; Elster and Burdette 2001; NessAiver 1997; McRobbie 2003)
MRI consists of applying the NMR principle to construct an image representing the proton density of different tissue voxels. Many of those protons are water protons such that MRI is particularly well suited for imaging soft tissue structures. The frequency of the proton MR signal is proportional to the magnetic field to which they are subjected during relaxation. An image can be made by producing a set of well-calibrated magnetic field gradients in all directions so that a certain magnetic field strength can be associated with a given location. In the longitudinal direction, a slice selective excitation is induced by applying a magnetic field gradient, a so-called slice selection gradient. This way, Larmor frequency and RF excitation fulfill the resonance condition in a specific axial slice such that only the magnetization of that slice is excited and contributes to the signal. In one of the transversal directions, phase encoding is applied with different phase-encoding gradients between RF excitation and readout. This is achieved by briefly switching on a magnetic field gradient in that direction when neither excitation nor readout is performed. This additional magnetic field gradient will induce, differences in spinning rate such that when the gradient is switched back off again, proton spinning is dephased along this direction and the signal is phase encoded. Finally, frequency encoding is applied along the other transversal direction by applying a readout gradient. This way, the frequency of the signal during readout is dependent on the spatial location in that direction. The signal of all voxels from the selected slice is recorded simultaneously in the so-called k-space by performing sequentially different phase-encoding steps. This k-space represents the Fourier transform of the MR image. The central part of k-space covers the lower spatial frequency range of the image representing the contrast and signal-to-noise content, while the outer part of k-space contains the high frequencies or resolution content. In case of contrast-enhanced MRI, the central part of k-space could be sampled first using a more centric approach, while for very fast scan techniques, spiral-like trajectories could be considered instead of the line at a time strategy. Multi-slice imaging and exploiting k-space symmetry can further reduce the acquisition time substantially.
During the phase encoding, phase is also encoded outside of the field of view (FOV), such that structures or body parts that are positioned outside of the FOV but within the encoded image slice contribute to the image and can cause aliasing artifacts in the phase-encoding direction. Other artifacts that mainly propagate in the phase-encoding direction are motion artifacts due to the movement of spins between the phase encoding and signal reading. During movement through the magnetic gradient field, spins change frequency and thus acquire an additional phase shift causing a spatial mismapping of the signal of these protons. In the frequency-encoding direction, the pace of spatial encoding and sampling is such that physiological motion only causes limited spatial blurring in that direction.
In contrast, chemical shift artifacts can arise in the frequency-encoding direction. The chemical bounding of protons causes a shift in their precession frequency due to magnetic shielding provided by the electron shell. For the same field strength, protons bound to water have higher resonance frequencies than protons bound to lipids. Therefore, spatial encoding by the frequency-encoding gradient will shift fat relative to water in the frequency-encoding direction resulting in dark and/or white band at the interfaces between water and fat. This chemical shift artifact can be reduced by suppressing the signal from fat using saturation or inversion recovery techniques. Another method to minimize chemical shift artifacts is to use a wider receiver bandwidth, such that the bandwidth for each pixel is wider and thus a given chemical shift corresponds to fewer pixels. Drawback of wider receiver bandwidth is however a lower signal-to-noise ratio.
5.2 MR Imaging Sequences (Bitar et al. 2006; Boyle et al. 2006; Poustchi-Amin et al. 2001)
In standard clinical setting, parameters of MR sequences are chosen such that images are generated with a contrast depending on the proton density, and thus tissue composition, or on the differences between T1- and/or T2-relaxation times between different tissue types. To understand how contrast is generated, one needs to be aware that after RF excitation, signal intensity of the transversal magnetization decays very fast due to T2 relaxation. This is called the free induction decay (FID) of the signal. Due to local microscopic magnetic field inhomogeneities and chemical shift effects, the signal actually decays much faster with a shorter effective time constant T2*. Therefore, an echo of the original signal is generated and measured. The time between initial excitation RF pulse and the echo signal is the echo time TE and one of the key sequence parameters together with the repetition time TR which represents the time between two consecutive RF excitation pulses. In general, standard MRI sequences can be subdivided in three classes depending on how the echo of the original signal is generated and how the readout of the signal is performed.
5.2.1 Spin Echo (SE) Sequence
Following the excitation pulse, the net magnetization in the transverse plane will start to dephase due to T2 relaxation and signal intensity will drop very fast. A short time after the excitation pulse, a 180° pulse is transmitted causing the spins to rephase and the signal to rise again. This signal is sampled and called an echo of the initial net transversal magnetization. Echo time is twice the time between the initial excitation RF pulse and 180° RF pulse. The initial excitation pulse used to induce a flip angle (FA) of 90°; however, smaller or larger flip angles are used as well. Advantage of a SE sequence is the strong signal which is compensated for local field inhomogeneities and different chemical shifts and is therefore less susceptible to artifacts. Rephasing however requires longer minimal echo times due to the additional 180° pulse and longer repetition times for an acceptable signal. This will increase total scan time and therefore the risk for motion while a larger amount of RF energy will be deposited in the body. In order to reduce acquisition time, multiple echoes are generated after a single excitation pulse using an echo train (Harms et al. 1986). The sequences are called turbo spin echo (TSE) or fast spin echo (FSE) sequences. The number of echoes is called the turbo factor, and measurement time is reduced by this factor compared to standard SE imaging. However, contrast in fast spin echo needs to be described differently compared to standard spin echo sequences since multiple echoes are generated at different echo times. From these echoes, the ones corresponding to the central part of the k-space will determine image contrast, and the time between these echoes and the excitation pulse is called the effective TE.
Since a 180° rephasing pulse is used for the echo, SE and FSE sequences are insensitive to microscopic magnetic field inhomogeneities caused by iron deposition or specific tissue composition. Therefore, real T2 weighting is only possible by this kind of sequences, excluding T2* contrast and opposed-phase imaging. On the other hand, SE and FSE sequences generate a strong signal compensated for local field inhomogeneities and therefore less sensitive to artifacts. Examples of T2 weighting can be found in Figs. 5.2 and 5.3.
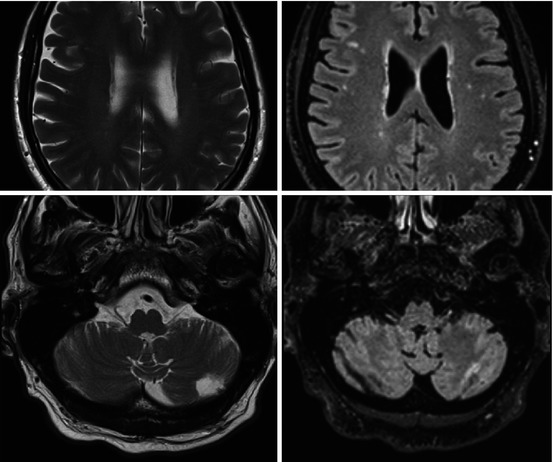
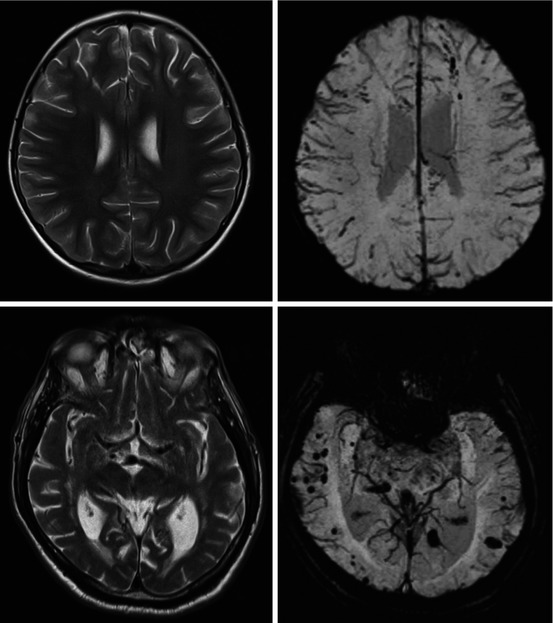
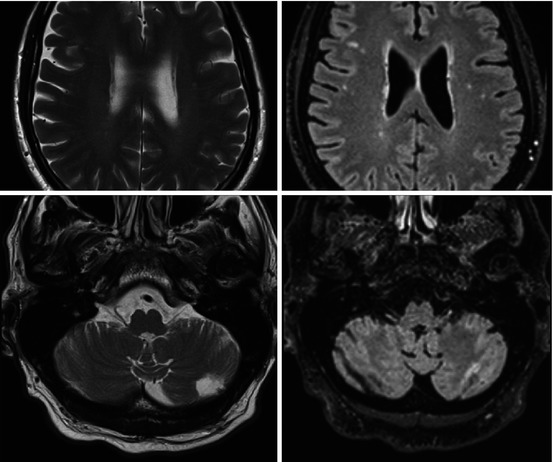
Fig. 5.2
T2-weighted TSE (left column) and FLAIR (right column) brain imaging. First row: subcortical white matter hyperintensities better visible on FLAIR compared to T2. Second row: cerebellar infarct visible on T2 TSE which can be missed on FLAIR
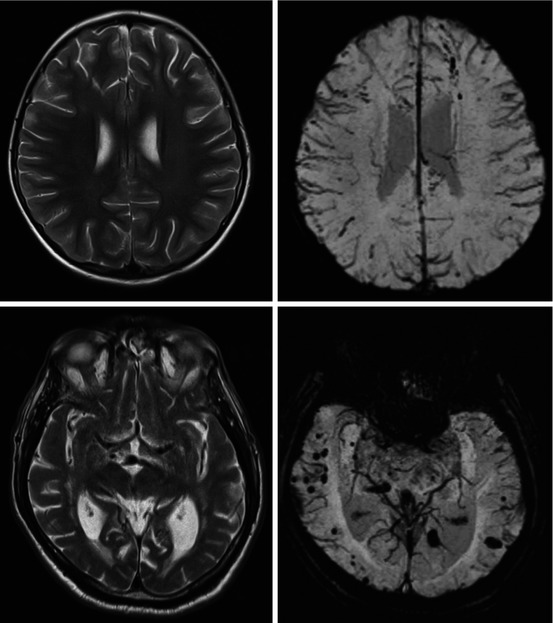
Fig. 5.3
T2-weighted TSE (left column) and SWI (right column) brain imaging. First row: diffuse axonal injury in an 8-year-old girl – multiple hypointense focal lesions on SWI not visible on T2 TSE, secondary to susceptibility from blood products after trauma. Second row: normotensive demented patient with cerebral amyloid angiopathy – multiple “black dots” on SWI in subcortical white matter in parietal and occipital lobes
5.2.2 Gradient Echo (GE) Sequence
GE sequences differ from SE sequences in the way the echo is formed. GE sequences create an echo by reversing the polarity of the readout or frequency-encoding gradient. A gradient pulse directly after the RF pulse enhances dephasing of spin frequencies such that the FID occurs considerably faster than under normal conditions. By reversing the polarity of the gradient, dephasing of the spins is reversed and spins start rephasing till at a certain moments all spins are in phase again and the echo is generated and measured. This gradient polarity reversal technique can work much faster than a 180° pulse for rephasing spins. This technique can be combined with a partial FA, which means smaller than 90°, making this approach very suitable for fast imaging sequences. Since a partial FA decreases the amount of magnetization flipped into the transverse plane, longitudinal magnetization is recovered faster, allowing a shorter TE and TR and thus decreased scan times while preserving image quality and limiting RF deposits. On the other hand, GE sequences are susceptible to microscopic magnetic field inhomogeneities and chemical shift effects. GE imaging sequences therefore produce T2*-weighted images reflecting faster spin dephasing and signal loss due to both spin-spin interactions and magnetic field inhomogeneities and allowing opposed-phase imaging. Due to different resonance frequencies of water and fat, the signal intensity from a voxel containing both water and fat will oscillate, reaching a minimum when fat and water are out of phase and a maximum when they are in phase. If the echo is created with fat and water in phase, their signals will add up. When the echo is performed when they are out of phase, their signals will be subtracted. This way, water and fat can be separated. For spin echo sequences, these effects are not feasible since phase shifts due to chemical shift are canceled by the 180° refocusing pulse.
5.2.3 Echo-Planar Imaging (EPI) Sequence
EPI sequences are a very fast way of acquiring MRI data and form the basis for many advanced MRI applications such as diffusion, perfusion, and functional imaging. The excitation pulse is followed by a gradient echo train to continuously measure the MR signal and fill the k-space completely (single shot) or partially (segmented acquisition) within a single TR interval. The gradient echo train consists of fast oscillating gradients for frequency encoding in the readout direction and short gradient pulses for phase encoding. Therefore, a high-performance gradient system is required with short rise times to allow frequent gradient switching. Specific compositions of readout and phase-encoding gradients define the spatial encoding and the trajectory to fill up k-space. Several types of trajectories such as spiral trajectories can be compiled to optimize sampling of k-space and reduce scanning time. However, this often results in poorer spatial resolution. Spatial encoding of EPI sequences is sensitive to magnetic susceptibility and can be disturbed by gradient imperfections. Increasing the scan time and using segmented rather than single-shot sequences can reduce artifacts. Due to the narrow readout bandwidth in the phase-encoding direction, chemical shift artifacts are possible in this direction requiring fat signal suppression.
The contrast generated with an EPI sequence is determined by the echo sequence and additional contrast-related gradient pulses. Both SE-EPI and GE-EPI are available.
5.3 MR Imaging Contrasts
The possibility of performing MRI with different image contrasts often results in considering MRI as a multimodal imaging technique or at least as multispectral imaging. Different contrast mechanisms are available such as proton density contrast and contrast based on relaxation along and perpendicular to the static magnetic field; chemical shift contrast of particular use for molecular imaging; motion-weighted contrast for imaging blood flow, tissue perfusion, and diffusion; susceptibility-weighted contrast used for functional MRI; and other advanced contrast mechanisms such as magnetization transfer.
5.3.1 T1, T2, and PD-Weighted Image Contrasts (Gibby 2005; Pooley 2005; McRobbie 2003; NessAiver 1997)
In case of SE sequences, the effect of T2 relaxation on the image is controlled by TE, while the impact of T1 relaxation is dependent on TR. For a sequence with a short TR and very short TE, spins will undergo very limited dephasing due to T2 relaxation, and without T1 relaxation all tissue types would generate a similar MR signal. However, the short TR will not allow the net magnetization in the longitudinal direction to recover fully between two RF excitation pulses. The recovered longitudinal magnetization will depend on the T1 relaxation time of the underlying tissue type. Fat for instance with a shorter T1 relaxation time will have a larger net longitudinal magnetization restored than water with a longer T1 relaxation time. Consequently, the transversal magnetization generated during the next RF excitation pulse will be higher for fat than for water, and therefore, the contribution of fluids to the overall signal will be lower. In this case the MR signal is T1 weighted, meaning that contrast is dependent on T1 relaxation differences between tissues where tissues with longer T1 relaxation times contribute less to the signal and therefore appear darker in the MR image.
If the TE of a SE sequence is prolonged, dephasing of spins due to T2 relaxation will be more pronounced and will depend on the differences in T2-relaxation times between tissue types. If a longer TE is combined with a relatively long TR, full recovery of the net longitudinal magnetization is obtained for all tissues and tissue differences in T1 relaxation are canceled out. Consequently, the transversal magnetization generated during the next RF excitation pulse is the same for all tissues such that the MR signal will be dependent only on differences in T2 relaxation and the MR image contrast will be T2 weighted. Water for instance has a long T2-relaxation time and will still show some phase coherence in case of a long TE, while most other tissues will have dephased much more and will contribute less to the MR signal. Hence, water will appear bright in a T2-weighted image, while other tissue types will have lower intensities (see Figs. 5.2 and 5.3).
If a sequence combines a short TE with a long TR, T2 relaxation will have a very limited impact on the image contrast, while the net longitudinal magnetization will have recovered for most tissue types. Thus, the MR contrast will be dominated neither by T1 relaxation nor by T2 relaxation but will depend only on the proton density (PD) of the different underlying tissue types. A tissue type with a high proton density such as water will produce a high MR signal and appear bright, while tissues containing few protons will generate a low signal and appear dark.
SE sequences are especially suited for acquiring images of the same slice with different contrast. Generating a second echo with a longer TE within the same TR interval and with the same phase encoding will generate a PD-weighted image for the echo with the short TE and a more T2-weighted image for the echo with longer TE. This way, a T2- and PD-weighted image of the same slice position can be acquired without increasing overall acquisition time. Specifically for FSE sequences, a short TR for T1-weighted sequences limits the echo train length. Therefore, FSE sequences are preferably used for T2-weighted imaging.
Contrary to SE sequences where the FA is mostly fixed to 90°, GE sequences use a range of flip angles and contrast is determined mainly by FA and TE. If FA decreases, T2* weighting is favored since the residual longitudinal magnetization will be higher and the recovery of longitudinal magnetization for a given T1 and TR will be more pronounced. A high FA and short TE will generate T1 weighting, while a medium FA and short TE corresponds to PD-weighted contrast.
Given these types of contrast, it is not easy to decide on the best image contrast for a given pathology. In general, T1-weighted contrast allows a clear delineation of anatomical brain structures, while for brain pathologies involving edema, T2- or PD-weighted contrast could be preferred (Fullerton 1984).
5.3.2 Inversion Recovery (IR)
Inversion recovery (IR) sequences are frequently used to generate T1-contrast-weighted images. IR sequences can be considered a standard sequence preceded by a 180° excitation pulse in the longitudinal direction. This 180° excitation pulse will induce T1 relaxation that takes twice as long as for a standard sequence. No T2 relaxation will occur since no transversal magnetization component is generated. After a specific time interval of T1 relaxation, called the inversion time TI, a standard sequence is applied to generate an image. Because of this preceding 180° excitation pulse, one can make better use of differences in T1 relaxation between various tissues to create higher T1-weighted image contrast. This is however at the expense of longer acquisition times compared to standard T1-weighted sequences. TR time is now defined as the time interval between two 180° excitation pulses in the longitudinal direction. Since TE is relatively short compared to TI, image contrast of IR sequences is mainly determined by TI. Choosing this TI appropriately will suppress the signal from specific tissue types. If for instance the standard sequence part of an IR sequence is started at the time the longitudinal magnetization vector of fat or cerebrospinal fluid (CSF) crosses the zero line, no magnetization can be flipped into the transversal plane and therefore no signal can be generated for this tissue type. In case of fat, this type of IR sequence is called short TI inversion recovery (STIR) (de Kerviler et al. 1998) and is very effective in those cases where fat signal interferes with the given pathology such as the detection of water in fatty tissue in case of inflammation in the joints or infiltrating tumors in bone marrow. The IR sequence for suppressing the signal of CSF is called fluid-attenuated inversion recovery (Eastwood and Hudgins 1998; Simonson et al. 1996) (FLAIR) (see Fig. 5.2). This sequence is very useful for visualizing demyelinating diseases and to assess vascular white matter hyperintensities and infarctions.
5.3.3 Susceptibility-Weighted Imaging (SWI)
The uniformity of the static magnetic field B0 is slightly distorted by the magnetic susceptibility or magnetizability of substances that are present in the FOV. This magnetic susceptibility exhibited by a tissue or substance will result in signal loss (Wendt et al. 1988). In particular the magnetic susceptibility of oxygenated and deoxygenated blood differ, with oxygenated blood being diamagnetic and decreasing local field strength, while deoxygenated blood is paramagnetic and increasing local field strength. Protons in the tissue surrounding the blood vessels are affected by this microscopic field variation, resulting in a loss of phase coherence. This induces signal cancelation and a decrease in total signal intensity. Next to phase changes at blood/tissue interfaces, the difference in magnetic susceptibility between oxygenated and deoxygenated blood, although small, also induces T2* changes between the arterial and venous bed of the vascular system. Using 3D GE sequences, susceptibility-weighted imaging (SWI) picks up these subtle differences in magnetic susceptibility in order to detect bleedings and small iron depositions (Chavhan et al. 2009) (see Fig. 5.3) or as part of a multimodal imaging approach for neurodegenerative diseases (Haller et al. 2010) such as Alzheimer’s (Ramani et al. 2006) and multiple sclerosis (MS) (Langkammer et al. 2013; Tavazzi et al. 2007; Walsh et al. 2014).
5.3.4 Diffusion-Weighted Imaging (DWI) (Luypaert et al. 2001; Bammer 2003; Le Bihan et al. 2006; Huisman 2003; Hagmann et al. 2006)
Diffusion reflects the Brownian, random movement of water molecules at microlevel. This random motion is hindered by cell membranes, fibers, or large molecules such as proteins, while concentration of these obstacles depends on tissue type or pathology (tumor tissue, abscess, and edema). Measuring the mobility of water molecules therefore gives indirect information about the tissue structures in terms of cell density and granularity such that tissue types can be better characterized and differentiated.
The contrast of diffusion-weighted imaging (DWI) is based on the mobility of the extracellular water by adding diffusion gradients to the imaging sequence. The spins of immobile water molecules will dephase by the first gradient pulse but will rephase by the second gradient pulse such that no impact on the signal is observed. Contrary, the spins of water molecules moving along the direction of the diffusion gradients during the time interval between the gradients will not be rephased by the second gradient and will stay dephased compared to the spins of the immobile water molecules and thus the signal will decrease. The more water molecules diffuse during the time interval between the two diffusion gradients, the more they will remain dephased after the second gradient and the more the recorded signal will be weakened. Weakening of the signal is limited to diffusion along the direction of the gradients, so diffusion gradients are applied in three perpendicular spatial directions. The diffusion magnitudes calculated along these three directions are averaged to provide a measure for a global diffusion-weighted image called a trace image.
DWI sequences are usually T2-weighted ultrafast 3D SE-EPI sequences sensitized for diffusion by adding diffusion gradients symmetrically before and after the 180° rephasing pulse. Therefore, contrast of DWI images is both diffusion and T2 weighted such that a strong signal could correspond to diffusion restriction as well as T2 shine through of a T2-sensitive lesion. The diffusion gradient pulses are characterized by the b value which is essentially dependent on the gradient amplitude, gradient duration, and time interval between the two gradients. In clinical practice a T2-weighted image with a b value of zero is combined with a diffusion-weighted image with a high b value (see Fig. 5.4).
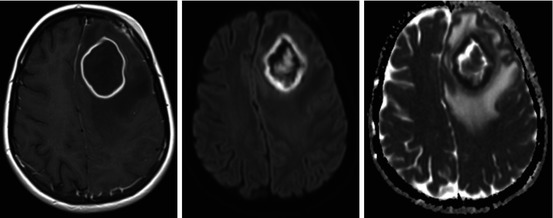
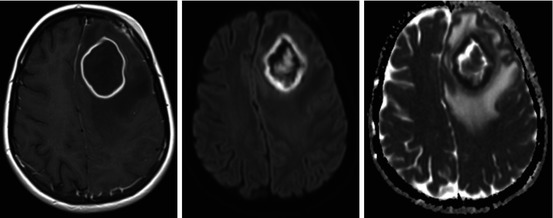
Fig. 5.4
Left frontal abscess after complicated sinusitis with ring enhancement on T1-weighted DCE-MRI with Ga-DTPA and restricted diffusion (high DWI and low ADC) in the border and central in the lesion
If DWI images are acquired for different b values, the amount and speed of movement can be quantified by the apparent diffusion coefficient (ADC) (Vandecaveye et al. 2008; Thoeny et al. 2012) (see Fig. 5.4). The ADC is calculated by fitting an exponential diffusion model to the signal intensities from images acquired with different b values. This way, only information on diffusion is retained and T2 shine through of T2-sensitive lesions is avoided. Whereas fast-moving molecules will quickly lose all of their phase coherence and signal intensity, even at low b values, slow-moving molecules will retain high signal intensities far into the higher ranges of b values. A solid tumor will show high signal intensity on a high b value image and low signal intensity on the corresponding ADC map, whereas apoptosis or necrosis will commonly appear as areas of low signal intensity on high b value images with high signal intensity on the corresponding ADC map.
This way, post-therapeutic changes induced by radiation therapy or chemotherapy generate a high signal intensity (edema) or low signal intensity (necrosis) on high b value images but generally show high signal intensity on corresponding ADC map. ADC information proves clinically relevant for other brain pathologies (Schaefer et al. 2000) such as degenerative (Vitali et al. 2011; Kim et al. 2011; Horsfield and Jones 2002) (Creutzfeldt-Jakob’s disease) or inflammatory pathologies (MS) (Tavazzi et al. 2007).
5.3.5 Perfusion-Weighted Imaging (PWI)
Generally, two approaches can be applied for perfusion-weighted MRI. The first approach uses endogenously generated contrast, while the second approach relies on the intravenous administration of MR-sensitive contrast agents. PWI information can be used to quantify brain blood volume and extract temporal information about brain blood flow on the level of microcirculation.
Arterial Spin Labeling (ASL)
Arterial spin labeling (Detre et al. 1992; Williams et al. 1992; Ferré et al. 2013; Laar 2008) is a PWI technique that does not require exogenous contrast agents. It uses the intravascular water in the arterial blood compartment as endogenous tracer. Water molecules are magnetically labeled by applying locally a 180° RF pulse to invert the longitudinal magnetization of arterial blood upstream just below the region of interest. With a time delay called the transit time TI, this arterial blood, tagged with an inverted net magnetization, will flow into the region of interest and act as a freely diffusible paramagnetic tracer. The inflowing inverted spins of the arterial water molecules will start to exchange with water molecules in brain tissue, reducing tissue magnetization and consequently weakening MR signal and image intensity. Next to this tag image, a reference image is acquired by repeating the sequence without tagging the hydrogen nuclei of the intravascular water. Reference and tag image are subtracted to provide a perfusion image, reflecting the amount of arterial blood entering the region of interest during the TI interval.
Major drawback of ASL is the rapid T1 relaxation and therefore limited lifespan of the tracer. Since a TI interval is required for blood to flow from the labeling location to the region of interest, labeled water within the arterial blood is decaying and labeling efficiency is decreased depending on the spatial position of the labeling region, blood flow, and vessel geometry. This can make ASL particularly challenging for studying cerebral vascular pathologies with limited blood flow.
Because ASL techniques require image subtraction and the flow-related signal is only a few percent of the tag and reference signal, motion can significantly degrade image quality and lead to large errors of the cerebral blood flow estimation. Ultrafast imaging sequences, such as EPI and FSE, or interleaved acquisitions of reference and tag data, provide higher SNR data and can significantly attenuate the effects of motion.
Techniques for ASL perfusion imaging can be sorted into two categories. The first category, continuous ASL (CASL) (Detre and Alsop 1999), uses a flow-driven continuous inversion technique where a constant RF pulse is applied off-resonance in the presence of a constant labeling gradient along the flow direction of the arterial blood. The frequency offset of the RF pulse is determined by the gradient strength and the desired distance from the inversion plane (called the labeling plane) to the region of interest. As arterial blood flows along the direction of the labeling gradient, the spins of arterial water experience a frequency sweep and are being inverted as they pass through the labeling plane. Once inverted, these water molecules will flow into the region of interest and influence the magnetic properties of the vascular bed of the target tissue.
The CASL approach requires arteries in a fairly straight segment along the labeling gradient and a sufficiently high blood flow such that frequency sweep is large compared to the relaxation time of arterial blood and small with respect to the amplitude of the RF pulse. Therefore, larger gradient strengths are required for smaller arteries with lower blood flow. Applying off-resonance RF pulses continuously during CASL causes saturation of protons bound to macromolecules (proteins, membranes…) as their T2 is very short, giving a very wide frequency band. The permanent exchanges between the unbound protons in water close to the macromolecules and protons bound to these molecules result in a magnetization transfer (MT) from the bound to unbound protons, reducing the observed MRI signal from the unbound protons in tissue and affecting quantification. To overcome this effect, a distal labeling is performed in the control experiment to produce identical MT effects. A small separate labeling coil, placed on the neck to label the common carotid arteries, can also avoid this effect since the FOV of the labeling coil is confined to the neck region and does not reach the brain, thus eliminating off-resonance effects, with the added benefit that it also greatly reduces the RF power deposition compared to a volume coil. Substantial RF power deposition in the body during CASL may be of concern for the routine use at high magnetic field strengths.
Next to CASL, pulsed arterial spin labeling (PASL) (Edelman et al. 1994; Kim 1995; Wong et al. 1997) can also be used as a technique for tagging water in arterial blood. Unlike CASL, where arterial blood is continuously inverted in a thin labeling plane, PASL techniques rely on a short RF pulse for inverting magnetization of all the water molecules contained in a thick region or slab proximal to the brain. Following this inversion, blood from this slab flows into the region of interest during an inflow time TI and interacts with the non-inverted water of brain tissue, after which the image is acquired. The control image is acquired in the absence of the slab-selective inversion.
Advantages of PASL over CASL (Wong et al. 1998) are the small MT effects and the lower RF power deposition due to the use of short RF labeling pulses, compared to the long continuous pulses used in CASL. The latter may become an important issue at high magnetic field strengths, such as 7 T and above. The major advantage of CASL techniques is the higher SNR compared to PASL, combined with a higher labeling efficiency because the labeling plane can, on average, be placed closer to the region of interest compared to the thick labeling slabs required in PASL. A post-labeling delay between end of the labeling interval and start of image acquisition can be included in the sequence. If this delay is longer than expected TI, all labeled water will have entered the region of interest prior to image acquisition, and concentration of labeled water in the arterial vessels will be minimized to avoid overestimation of blood flow and vascular volume artifacts. For PASL, determining the temporal width of the volume of labeled water is not straightforward since it depends on the spatial extent of the inversion slab, the arterial blood flow, and the geometry of the proximal vessels, while for CASL, estimating the TI is more evident.
A hybrid approach that simulates CASL using many short pulses termed “pseudo continuous” or “pulsed continuous” ASL (pCASL) (Dai et al. 2008; Wu et al. 2007) combines these two approaches to provide better sensitivity and ease of implementation for body coil transmitters. Several methods also exist for spatially selective labeling, uniquely allowing the perfusion distribution of single arterial territories to be measured. Velocity-selective labeling (VS-ASL) (Wong et al. 2006) has also been explored as a means of eliminating arterial transit time dependence. With this technique, the tagging scheme selectively saturates flowing spins with no spatial selectivity. This is accomplished with an RF and gradient pulse train that effectively dephases the MR signal from protons that are flowing faster than a specified cutoff velocity while rephasing the signal from slower flowing protons. The advantage of this approach is a small and uniform transit delay for the delivery of the tagged blood to the tissues of interest.
Clinical applications of ASL have demonstrated the challenge of optimizing labeling timing, in particular the post-labeling delay or TI time, to allow all labeled blood to enter the microvasculature while not losing too much signal to T1 decay because of delay times. Several studies of ASL in acute stroke have been reported revealing that ASL underestimates flow in many of the cases (Chalela et al. 2000; Siewert et al. 1997), and imaging performed with a longer wait before acquisition, either post-labeling delay or TI, was more consistent with other findings (Yoneda et al. 2003; Wolf et al. 2003).
ASL can provide either baseline measurements for between group comparison or serial measurements in individual subjects. As a serial measure, it can be used for fMRI with shorter timescale changes within a single scanning session or for changes on longer timescales to image the changes in the brain with normal aging (Wang et al. 2008).
On the other hand, the repeatability and noninvasiveness of ASL makes this technique suitable for monitoring neurological effects of pharmacologic agents and drug administration (Wang et al. 2011; Chen et al. 2011; Detre et al. 2009; Nordin et al. 2013), although vascular and neuronal effects of ASL cannot be separated. The temporal stability of ASL makes it also valuable for the study of brain activation changes over longer timescales (Wang et al. 2005). Activation studies with ASL may improve understanding of a number of important neural functions, such as memory consolidation or emotional state (Brown et al. 2007; O’Gorman et al. 2008).
In terms of clinical applications for ASL, stroke and cerebrovascular diseases are logical indications, but ASL also offers several important advantages for tumor blood flow assessment (Warmuth et al. 2003). Because of its insensitivity to vascular permeability, blood flow measurements are not confounded by permeability factors as in dynamic susceptibility contrast. Therefore, absolute blood flow quantification is feasible such that tumor blood flow can be used as an indicator of tumor grade (Järnum et al. 2010; Schlemmer et al. 2009; Wolf et al. 2005) and tumor blood flow values can be compared throughout the duration of therapy. Since ASL is insensitive to susceptibility variations that can result from surgical interventions or hemorrhage and high-resolution anatomical information can be acquired in the same scanning session, it can be a useful pre- and postoperative tool.
In the context of dementia, ASL may be useful in the early detection of AD-related changes in subjects with mild cognitive impairment (Alsop et al. 2000, 2008, 2010; Wolk and Detre 2012; Johnson et al. 2005). Loss of tissue and metabolic function are important hallmarks of dementia. In Alzheimer’s disease, loss of tissue is detected in the medial temporal lobes including the hippocampus. Metabolic imaging has demonstrated functional decreases in the posterior-inferior temporal cortex, the superior temporal-parietal association cortex, the posterior cingulated cortex, and in advanced cases also the frontal association cortex. ASL perfusion MRI to patients diagnosed with AD has found similar regions of decreased function while blood flow was relatively preserved in the medial temporal, superior temporal, and inferior frontal regions.
Dynamic Susceptibility-Weighted Contrast-Enhanced MRI (DSC-MRI)
DCS-MRI (Sourbron 2010; Ostergaard et al. 1996a, b; Verma et al. 2013) uses an intravenous bolus injection of a paramagnetic tracer such as a gadolinium-DTPA chelate (diethylene triamine pentaacetic acid) combined with dynamic imaging technique which measures the signal changes induced by the tracer in the tissue as a function of time. Using T2*-weighted ultrafast EPI sequences, data sampling is performed on a timescale smaller than typical tracer transit times through the capillary bed. This way the tracer is essentially intravascular during the first pass of the bolus and pure perfusion weighting is achieved. With these sequences, T2* signal loss due susceptibility effects of the contrast agent during first pass is picked up, and this signal decrease is used to compute the relative perfusion of that region (see Fig. 5.5). The DSC-MRI hemodynamic parameters are typically obtained from the time susceptibility curve while eliminating tracer recirculation effects. Typical quantitative parameters are time to peak (TTP), mean transit time (MTT), cerebral blood volume (CBV), and cerebral blood flow (CBF = CBV/MTT).
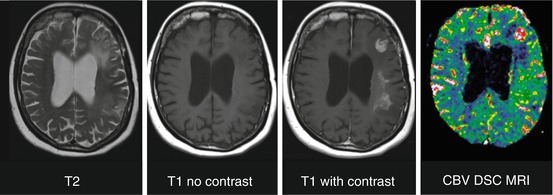
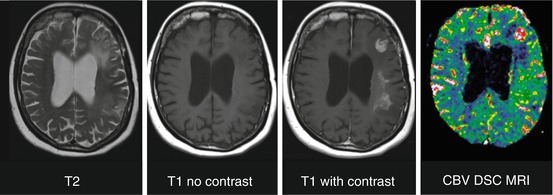
Fig. 5.5
T2 hyperintense lesion in left frontal lobe and left frontoparietal white matter in a patient after resection and radiotherapy of low-grade astrocytoma. Enhancement and elevated CBV indicating degeneration to high-grade astrocytoma
In comparison to other MR perfusion techniques (Weber et al. 2003), DSC-MRI has several advantages, such as better SNR, shorter scan times, ease of use, and greater availability for commercial scanners. These advantages make DSC-MRI the most widely used perfusion technique for the brain, although it is increasingly being challenged in recent years because of quantification issues (Calamante 2009). Since the technique remains highly sensitive to susceptibility artifacts, its application in patients with hemorrhages, calcifications, or surgical clips is limited. The administration of a contrast agent also makes DSC-MRI more invasive compared to ASL and difficult to conduct in patients with renal impairment. On the other hand, T1 shortening of the blood due to the administration of a contrast agent, such as gadolinium-DTPA, prohibits further ASL acquisitions. Contrast media in larger vessels in the field of view can interfere with the blood flow measurements, whereas this effect is small for ASL. In case of blood-brain barrier breakdown, the tracer extravasates into the interstitial space causing an accelerated T1 relaxation of the surrounding brain tissue, loss of T2* weighting, and potential errors in the calculation of the hemodynamic parameters.
Dynamic Contrast-Enhanced (DCE) MR
While T2* effects are significantly stronger for intravascular paramagnetic contrast media such as gadolinium-DTPA, T1 relaxation of water molecules of surrounding brain tissue is strongly accelerated as well. This signal can be picked by T1-weighted DCE-MRI sequences using EPI or rapid gradient echo techniques (Cha 2003). Although in the brain, DCE-MRI signal changes remain weaker than DSC-MRI, technological advances leading to shorter echo times and higher field strengths have improved the DCE-MRI data quality. While DCE-MRI has less temporal resolution than DSC-MRI, it has some advantages compared to DSC-MRI such as better spatial resolution that allows a better characterization of the vascular microenvironment of the lesion and robustness to the presence of susceptibility artifacts.
Specific indications are vessel wall imaging and imaging of inflammation where an increased vascular permeability is expected, imaging of neovascularization corresponding to an increased vascularity, and fibrous tissue characterization which induces an increased distribution volume (Bley et al. 2005; Kerwin et al. 2003; Desai et al. 2005; Yuan et al. 2002; Wasserman et al. 2002; Kramer et al. 2004).
5.3.6 MR Angiography (MRA)
MRI sensitivity to movement in terms of spatial encoding perturbations and artifacts can be used to develop vascular imaging approaches based on signal changes that are linked to flow. Techniques such as time-of-flight MRA (TOF MRA) or phase-contrast MRA (PC MRA) apply endogenous vascular contrast, while contrast-enhanced MRA exploits the relaxation properties of exogenous contrast agents to visualize vascular structures (van Laar et al. 2006; Ozsarlak et al. 2004).
MR angiography techniques are generally T1-weighted echo-gradient-based sequences. When endogenous vascular contrast is used, strategies are implemented to suppress the background signal represented by the stationary tissue such that vascular signal is favored over that of the surrounding tissues (Foo et al. 2005).
TOF MRA generates contrast between blood flow and stationary tissue by changing the magnitude of magnetization such that moving spins generated a high signal while the signal of stationary tissue spins will be diminished. Using repetition times much shorter than the relaxation times of brain tissue, spins in a predefined slice do not fully recover after each repetition and the MR signal decreases with the number of excitation pulses until equilibrium is reached. Moving spins that flow into this slice are not saturated and therefore have a greater signal, creating contrast between saturated stationary tissue and blood. Drawbacks are the long acquisition time and saturation of in-plane blood vessels, while the signal of stationary tissues with short T1 relaxation time such as fat, hematoma, or thrombus can be poorly suppressed.
PC MRA on the other hand generates contrast between blood flow and stationary tissue by changing the phase of magnetization such that the phase of moving spins is shifted relative to the phase of stationary spins. This technique uses a bipolar flow encoding gradient of given intensity to dephase spins in the transversal direction in proportion to their velocity in the direction of the gradient. This generated phase difference between stationary and moving spins can be picked up to derive contrast between flowing blood and brain tissue.
Next to TOF MRA and PC MRA, time-resolved ASL (Robson et al. 2010) has been developed as a noninvasive alternative to angiography.
In the case of CE MRA (Zhang et al. 2007a), signal differences are achieved not only by employing the appropriate sequence parameters but also by intravenously injecting a contrast agent into the vascular system to selectively shorten the T1 relaxation of the blood. Using a T1-weighted imaging sequence during the first pass of the contrast agent, the shortened T1 relaxation of blood causes the blood to give rise to a very large signal producing a very high contrast between arteries and surrounding tissues and veins.
While MRA using endogenous contrast can be disturbed by complex or turbulent flows, the blood signal of CE MRA is minimally affected by dephasing caused by complex flows and susceptibility variations. The key factor for CE MRA signal is to acquire data at the right moment, during the passage of the contrast agent bolus. Because the CE MRA techniques are relatively insensitive to this signal loss, they provide high-quality images with fewer artifacts than the non-CE methods. Because effects of saturation are minimal, large fields of view can be imaged with high SNR to demonstrate large vascular areas in a short acquisition time. The short imaging time permits acquisition in a single breath-hold interval, providing high-quality images even in areas affected by respiratory motion.
5.3.7 Advanced Functional MRI Techniques
Next to the MRI techniques which were mentioned above and which are used extensively in a clinical setting, other MRI techniques will be discussed in brief. These methods are mainly used for addressing scientific questions rather than for clinical diagnosis or therapy evaluation. However, for specific brain pathologies, they could move from a research setting into clinical tools in the near future.
BOLD fMRI
Neuronal activity not only increases local oxygen consumption but also induces an even higher increase in local blood supply, due to neurovascular coupling. Therefore, neuronal activity is reflected by a higher oxygenated over deoxygenated blood ratio, which causes a local paramagnetic effect. This blood-oxygenation-level-dependent (BOLD) effect can be picked up by MRI as a weak, transient T2*-weighted signal rise, very similar to SWI. Since the BOLD contrast is poor, fast T2*-sensitive sequences with high SNR and sufficient temporal resolution are required for functional MRI (fMRI) studies. Disadvantages of using BOLD contrast for fMRI studies are related to motion artifacts caused by head motion, breathing, and cardiac pulsation and susceptibility artifacts causing signal distortion or signal loss at tissue interfaces with bone structures and air cavities. BOLD fMRI also maps neuronal activity using an indirect effect which can lead to imprecise localization of the activated zone due to spatial discrepancies between activated neurons and corresponding vascular variations in the oxy- over deoxy-hemoglobin ratio.
As an alternative to BOLD fMRI, ASL can be used to detect variations in perfusion after neuronal activation. Like BOLD, ASL only indirectly reflects neuronal activity by detecting vascular changes. However, it provides an absolute quantitative measure of cerebral blood flow (CBF) compared to BOLD, which is a complex function of a number of physiological variables, especially oxygen utilization, cerebral blood flow, and cerebral blood volume. Moreover ASL fMRI is less sensitive to baseline signal drift and low-frequency noise and may provide higher spatial specificity for neuronal activity. Several studies have shown that ASL measurements have decreased intersubject and intersession variability as compared to BOLD (Aguirre et al. 2002, 2005; Tjandra et al. 2005), possibly due to a more direct link between CBF and neural activity. On the other hand, SNR of ASL is lower while in general less imaging coverage and lower temporal resolution is provided than with BOLD fMRI. This is because of the acquisition of a tag and control dataset, the need to wait for tagged blood to flow into the imaging region and the need to acquire data before the tagged blood signal has fully relaxed. Therefore, ASL fMRI is particularly suitable for experimental paradigms with task repetitions at low frequency or with long stimulus durations (Wang et al. 2003). However, further development of single-shot ASL acquisition protocols (Wong et al. 2000; Günther et al. 2005) with whole brain coverage will keep on challenging BOLD fMRI, as these sequences provide similar temporal resolution and spatial coverage with improved temporal stability and without susceptibility artifacts. It has also been suggested to combine ASL with BOLD imaging, as ASL perfusion measurements can aid in the interpretation of the BOLD signal change and, when combined with BOLD, provide an indicator of oxygen utilization (Hoge et al. 1999).
In a research setting, fMRI is widely used for neuropsychological and cognitive studies. In task-based fMRI, specific tasks are performed during fMRI data acquisitions, such that the neuronal activity changes during task performance can be monitored. Acquisitions are repeated in time during a succession of different tasks following an activation paradigm. This activation paradigm comprises the task sequence and mode of repetition. It consists of at least a baseline task and other tasks, which only differ from the baseline task by a specific activity. Analysis is based on a statistical comparison of the signal variations measured in each pixel between different states of activation and thus relate to the differences between the two tasks.
Next to task-based fMRI, fMRI can also be used to study brain functioning while subjects are not engaged in any specific task. These resting-state fMRI or RS-fMRI studies monitor the functional brain activity during resting state as spontaneous low-frequency BOLD signal fluctuations (Dijk et al. 2010; Buckner et al. 2008). Interregional temporal correlations of these BOLD signal fluctuations provide estimates for the temporal correlation between neurophysiological measurements obtained in different brain areas and thus for resting-state functional connectivity (RSFC). This coherent activity of functionally related brain areas is an important feature of healthy brain functioning (Fox and Raichle 2007; van den Heuvel et al. 2009).
Since RS-fMRI is noninvasive and does not require cognitive task performance during image acquisition, its setup is substantially simpler than other functional neuroimaging methods. RS-fMRI detects multiple brain networks presenting consistent intercorrelations of low-frequency activity, including the primary sensorimotor network, the primary visual and extrastriate visual networks, the frontoparietal attention networks, and the default-mode network (DMN), which is directly associated with episodic memory retrieval, self-referential processes, social cognition, and mind wandering (van den Heuvel and Hulshoff Pol 2010; De Luca et al. 2006). Functional connectivity abnormalities in one or more of those networks may be found in psychiatric and neurological disorders, while showing significant correlations with behavioral changes and cognitive deficits (Broyd et al. 2009; Greicius 2008; Damoiseaux et al. 2012; Galvin et al. 2011; Kwak et al. 2010; Sheline et al. 2010; Cole et al. 2011; Anticevic et al. 2012). Given its feasibility and reliable and replicable results (Chou et al. 2012; Koch et al. 2010), RSFC abnormalities associated with the above disorders could have future potential as a disease biomarker in a clinical setting, both for diagnosis and treatment monitoring (Koch et al. 2012; Van Dijk and Sperling 2011).
Diffusion Tensor Imaging (DTI) MRI
While DW-MRI measures differences in water mobility without taking into account the direction of the displacements, DTI MRI detects motion of water molecules in different directions and can characterize the mobility in terms of anisotropic diffusion (fractional anisotropy), main diffusion direction, and preferred directions or restrictions (Hagmann et al. 2006). This is particularly of interest for nerve fibers since it can be applied indirectly to reconstruct nerve fiber trajectories. Since nerve fibers consist of axon bundles running in parallel with concentric layers of myelin-restricting transversal diffusion, water diffusion will preferably follow the direction of the fibers while being restricted in the direction perpendicular to the fibers. This causes anisotropic diffusion in white matter tissue of the brain. By performing diffusion-weighted acquisitions in different directions, a tensor can be determined characterizing the diffusion. This diffusion tensor can give information about nerve fiber lesions in white matter or spinal cord and allows tracking of the nerve fiber trajectories in the brain. The latter can provide valuable preoperative information for brain tumor resection or can be of diagnostic use (Stahl et al. 2007; Agosta et al. 2011; Horsfield and Jones 2002). Fiber tractography (Nucifora et al. 2007) can also be combined with fMRI to study the connectivity between functionally different brain regions and to analyze brain maturation and development in terms of myelinization.
The accuracy of fiber tractography is limited by possible fiber crossings in the same voxel leading to errors in fiber trajectories. Diffusion measurements in more different directions can increase the accuracy, albeit at the expense of longer acquisition times.
5.3.8 High-Field MRI
Increasing the magnetic field strength yields images with a better signal-to-noise ratio (SNR), a higher spatial resolution, shorter acquisition times, and a better contrast for tissue characterization (Schick 2005; Stafford and Challenges 2003; Panebianco et al. 2013; Mri and Quick 2011). In terms of relaxation times, T1 relaxation time increases, while T2-relaxation time decreases. Therefore, MRI sequences require appropriate adjustment for the slower longitudinal relaxation, while a shorter TE is needed to comply with the reduced T2-relaxation time.
Both TOF MRA (Nguyen and Yang n.d.) and ASL benefit from the intrinsically higher SNR and the lengthening of blood T1 relaxation providing better contrast between tissue and the circulating blood. Increased contrast between tissue and paramagnetic contrast agents will allow a reduced injection dose of these agents. Higher sensitivity to magnetic susceptibility has a positive impact on the detection of hemorrhages, first-pass perfusion imaging, and BOLD fMRI signal. In terms of chemical shift, the larger gap between the resonance frequencies of fat and water makes saturation of the fat signal much easier and more homogeneous.
Besides these advantages, high-field MRI has some limitations as well (Schick 2005; Stafford and Challenges 2003). Due to the shortened wavelength of the RF pulse, now being much shorter than the FOV, RF excitation occurs much more inhomogeneous. On the other hand, the increased magnetic susceptibility can be diminished by reducing the TE and making the sequence less T2* sensitive or by choosing segmented rather than single-shot sequences and increasing the bandwidth. Finally, during high-field MRI, the amount of RF energy deposited in tissue is increased significantly and needs to be addressed by increasing TR, limiting the number of slices, reducing the flip angle, or shortening the length of echo train.
In this context, it is also worthwhile to briefly mention parallel imaging (Glockner et al. 2005; Deshmane et al. 2012; Setsompop et al. 2008). Multiple small diameter coil elements can be combined in a phased array to record signals simultaneously and independently. Each coil element has a limited receiving range and a sensitivity profile that depends on the distance from the coil element. Therefore, the signal received by each coil element contains spatial data such as position coil position, reception level, and sensitivity level. This information can be combined with the gradient-induced spatial encoding to reconstruct the image. Since small diameter coils have higher signal-to-noise ratios than coils with larger diameters and noise between the different elements in the phased array is not correlated, the signal will have a better SNR than that one large coil.
Techniques combining the signals of several coil elements in a phased array to reconstruct an image are called parallel acquisition techniques. These techniques can be used to increase SNR, improve image quality for the same acquisition time, or reduce the acquisition time. The latter is particularly suitable for breath-hold sequences or for improving the temporal resolution of perfusion imaging (Oliver-taylor 2012), functional imaging, and dynamic imaging of movements while reducing flow and motion artifacts. Especially cardiac and abdominal MRI or MRA (Wilson et al. 2004) can benefit from this approach.
In terms of reconstruction, two approaches have been presented (Blaimer et al. 2004). The first approach combines the images from each coil to reconstruct the global image in the spatial domain (f.e. SENSE or sensitivity encoding) (Zhang et al. 2007b) and is the most widely spread in present commercially available parallel MR sequences, while the second approach combines the frequency signals of each coil in the Fourier domain (GRAPPA or generalized auto-calibrating partially parallel acquisition) (Hoge and Brooks 2008).
In the context of ultrahigh-field MRI (Wiesinger et al. 2006), parallel transmission techniques, where the independent RF pulses from an array of small coils can be combined to a more complex and adaptive RF pulse, offer multiple potential improvements in radiofrequency transmission (Katscher et al. 2003; Katscher and Börnert 2006). More advanced RF transmission can lead to shorter pulse duration and reduce energy disposition in the patient, while patient-specific inhomogeneities can be corrected for, especially in ultrahigh-field MRI. Therefore, advanced parallel acquisition techniques allow sequences of the echo-planar type and ultrafast gradient echo type, even at a very high field (Webb and Collins 2010; Harvey and Hoogeveen n.d.).
5.4 PET Basics
PET has extensively been used to explore a variety of physiological, biochemical, and pharmacological processes and to study aspects of the complex interaction of several neurotransmitter systems in the brain (Kenneth et al. 2002; Savitz and Drevets 2013; Smith and Jakobsen 2009). Because of the extremely low amounts of PET radiotracers (a compound labeled with a radionuclide) needed for imaging, its administration causes no pharmacological or functional changes in the physiology of the organism. Therefore, PET can measure nanomolar molecular concentrations and is highly sensitive and quantitative compared to MR-based techniques. The most challenging aspect is the development of suitable PET tracers for brain imaging which need to match a relatively small window of appropriate lipophilicity and molecular weight and affinity while demonstrating high target specificity and appropriate behavior in terms of metabolization.
A typical PET study involves the injection of a radiotracer into the venous blood stream of a subject. This radiotracer is delivered to the brain by the arterial flow. After crossing the blood-brain barrier, it might bind reversibly or irreversibly to its targets, which may either be neuroreceptors, transporter vesicles, or be metabolized by endogenous enzymes. If the tracer is inert, it will diffuse across the blood-brain barrier and will not be bound or trapped. In parallel to these biochemical processes, the radioactive label will decay, emitting a positron that annihilates through the simultaneous emission of two 511 keV photons in opposite directions. These photon pairs will be detected by the PET scanner within a predefined timing window (usually 6–10 ns) as a pair of coinciding detections. Therefore, PET is also being referred to as coincidence imaging. Over the total duration of the emission scan, data are acquired and corrected for physical effects such as attenuation, scatter, dead time, and detector response. These corrected data are binned into different time frames and each time frame is reconstructed using an analytical or iterative reconstruction algorithm. This way a three-dimensional image of the radiotracer distribution in the brain is generated at various time points after tracer injection. These PET data are denoted dynamic and represent the temporal and spatial distribution of radiotracer concentration in brain tissue. The temporal evolution of radiotracer concentration in individual brain voxels or regions can be visualized by a time-activity curve (TAC). These TACs form the basis for quantifying the physiological (e.g., blood flow) and/or pharmacological aspect (e.g., receptor-binding site density, enzyme activity) of the system of interest.
Appropriate in vivo quantification of molecular targets with PET imaging is relatively complex due to the fact that tracers are administered intravenously and not directly applied to the target tissue. Therefore, delivery of the tracer to the brain can be influenced by the local blood flow, free tracer concentration in the plasma, and peripheral tracer clearance due to metabolization and excretion. Moreover, total brain activity is measured with PET brain imaging, while often specifically bound, nonspecifically bound, and free tracer need to be separated to estimate the specific tracer kinetics.
In terms of mathematical analysis, brain uptake of a radioactive tracer is often described within the theoretical framework of compartments. Compartment modeling allows the description of systems that vary in time, but not in space. One of the assumptions for compartmental modeling is that there are no spatial concentration gradients within each department but only gradients in time. In fact, a compartment represents a unique state of the tracer and is defined as a space with separate uptake and clearance rate constants where the radioactive tracer concentration is assumed homogeneous. Rate constants of each compartment are assumed time invariant at least over the duration of the study and considered to be representative for the steady state of the system and the properties of the ligand. A compartment may have a physical analog such as the interstitial fluid compartment but can also be considered as a tracer being in bound or unbound state. Once the exchange paths between compartments have been specified, the mass balance for each compartment can be described as a set of ordinary differential equations where the tracer concentration in the vascular arterial compartment drives the model.
In the next paragraphs, brain PET perfusion imaging will be discussed, introducing elementary compartmental modeling. The basic kinetic blood flow model will be linked to a more general one tissue compartment model and extended to a two tissue compartment model. The latter will be considered for the quantification of [18F]FDG brain PET imaging. Finally, the potential to acquire PET information from multiple tracers simultaneously will be discussed.
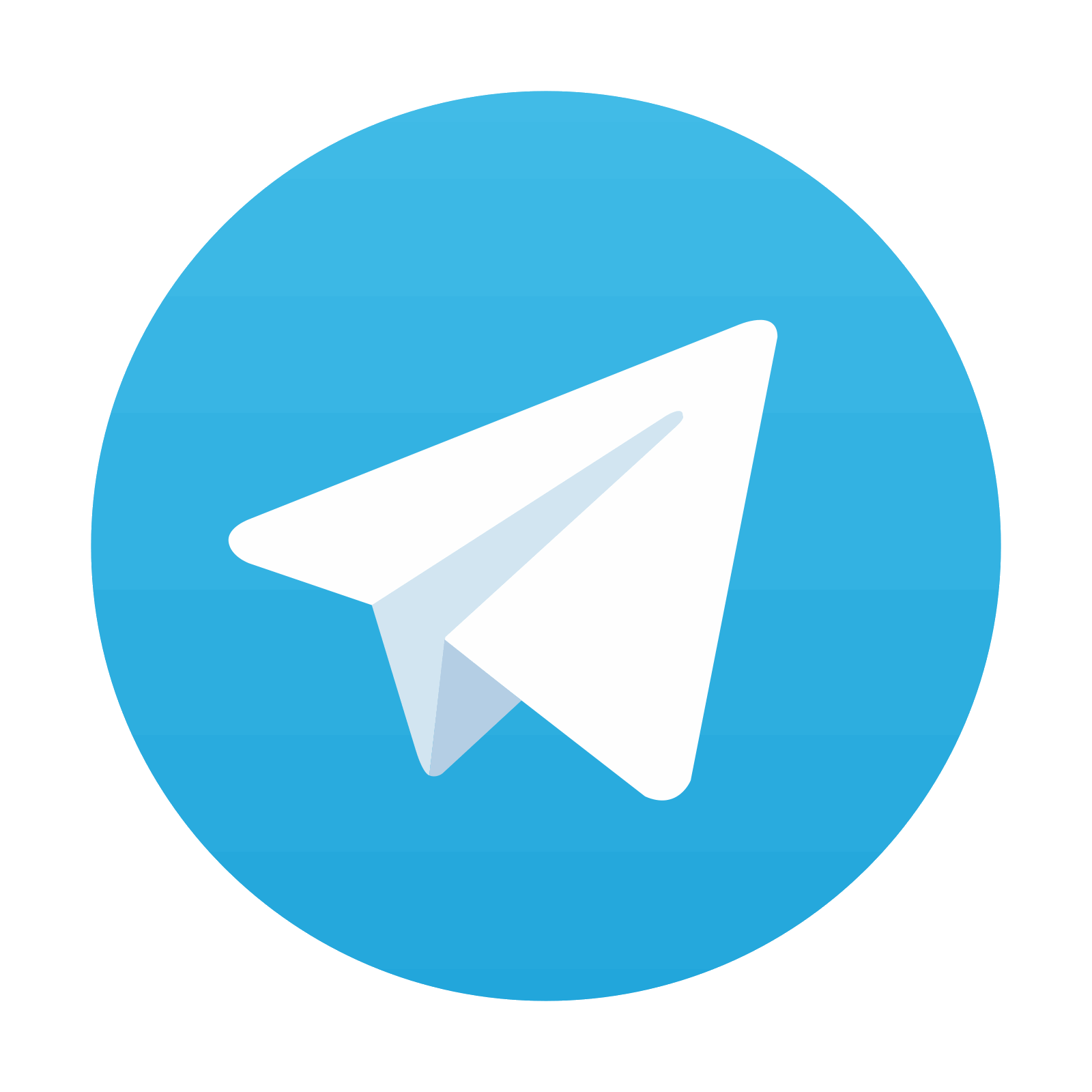
Stay updated, free articles. Join our Telegram channel
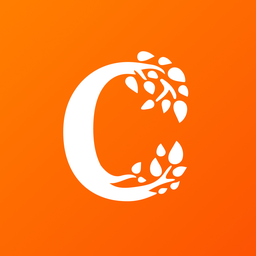
Full access? Get Clinical Tree
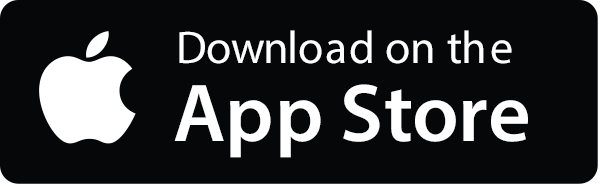
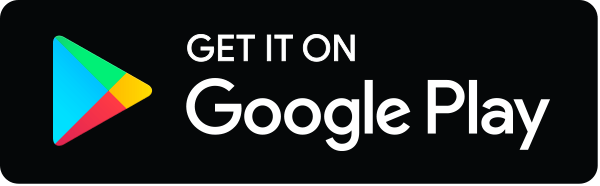