47CHAPTER 4
Patient Immobilization for SRS and SBRT
Stereotactic radiation therapy, in the cranium and in the body, rests on the idea that improved localization of target structures will permit the use of smaller treatment planning margins than those used in conventional external beam radiation therapy. These smaller target volumes will in turn allow higher fractional radiation doses to be delivered safely. To reduce planning margins, however, extreme care must be taken in patient setup and positioning for treatment. Effective immobilization is critical in minimizing intrafraction motion of the patient, which could result in catastrophic consequences in high-dose fraction delivery. In addition, stereotactic immobilization should be stable and relatively comfortable for long treatment times.
Early stereotactic radiosurgery (SRS) systems almost exclusively used rigid, invasive skull fixation systems that incorporated a stereotactic coordinate system in the frame. The use of image-guided radiation therapy (IGRT) has allowed the use of nonrigid, relocatable frames often very similar to those used for conventional radiation therapy, and expanded the scope of radiosurgery to targets throughout the body.
The process of immobilizing the patient is therefore tightly bound to the imaging process (see Chapter 5). During initial simulation, a standard immobilization system is generally used for a particular treatment site, depending on its location in the body, the rigidity of the target, and the presence or absence of intrafraction motion (Figure 4.1). When developing a new relocatable system, repeat imaging studies may be used to assess repeatability of setup. Simulation lasers may be used to mark the relative position of the patient in the frame.
During treatment planning, fiducials in the treatment frame may be used to generate stereotactic coordinates for the target. It is important, particularly for noncoplanar treatments, to account for the bulk of the frame when determining clearance of treatment beams.
It is critical, particularly for noncoplanar beams, to verify the safe delivery of treatment. This involves confirming clearance of the beams around the patient/immobilization device, use of fiducials to establish target location, and IGRT confirmation. If the position of the patient is found to have shifted in the frame, relocalization of the patient in the frame may be necessary before treatment.
INTRACRANIAL IMMOBILIZATION
Intracranial immobilization can be divided into two main types: invasive and noninvasive fixation. Invasive, rigid frames provide the most accurate localization, but require the entire process of immobilizing, scanning, planning, and treating the patient to be completed in a single day. Noninvasive, relocatable frames may provide nearly equivalent accuracy, in particular when combined with IGRT, and allow the planning process to be done over several days; they also allow for fractionated treatment. Both invasive and noninvasive immobilizations for cranial SRS are capable of 1 mm accuracy (1–3).
SRS was first developed in 1949 by Lars Leksell, a Swedish neurosurgeon, by adapting equipment used for cranial surgery (4). Today, the Leksell frame (Figure 4.2) is still in use for the Gamma Knife, a treatment unit designed specifically for intracranial radiosurgery. The frame is securely attached to the patient’s skull with screws to provide a rigid immobilization. The process is normally performed with patient in conscious sedation with local anesthesia. The frame also defines a stereotactic coordinate system, which is used to determine the tumor location and treatment geometry. The origin is at the patient’s right, posterior, superior corner, and the frame center is at (100, 100, 100). There is no negative number within the system, which eliminates possible errors with signs. After placement of the frame, a special helmet is used to take depth measurements at several points to develop a model of the patient’s skull and later to verify that the frame has not shifted before treatment. The patient is then imaged using either MRI or CT or both, with a fiducial box attached to the patient’s stereotactic frame. The lesions, skull boundary, and fiducials are all identified in the image, and used to design the radiosurgery treatment plan. During treatment, the patient with the stereotactic frame is locked to the treatment couch, and the plan parameters with respect to the frame are transferred to the Gamma Knife treatment unit so that the beams can be precisely delivered to the target.
48
FIGURE 4.1 Process map of immobilization in radiosurgery.
IGRT, image-guided radiation therapy.
The most recent Gamma Knife unit, the Icon (5), now allows for the use of noninvasive thermoplastic masks for immobilization, and cone-beam computed tomography (CBCT) imaging for pretreatment verification. This eliminates the need to perform the entire planning and treatment procedure in a single day.
FIGURE 4.2 Leksell head frame for radiosurgery.
Source: Image courtesy of Elekta.
Linac-based radiosurgery systems also initially adopted invasive neurosurgical head frames, using adapters to mount them to the linac treatment couch. Later linac-based radiosurgery systems used noninvasive head frames with bite-block systems to register the frame to the patient’s cranium (Figure 4.3). These systems could achieve comparable immobilization uncertainty to invasive frames, while allowing for fractionated delivery. Some of the frames incorporated an infrared fiducial array attached to the bite-block, which could be monitored continuously to ensure the patient did not move during treatment (2).
FIGURE 4.3 Noninvasive bite-block frame used for relocatable cranial immobilization. Fiducial array attached to the frame allows for continuous monitoring of patient motion.
Source: Image courtesy of Varian Medical Systems, Inc. All rights reserved.
49More recent linac-based systems and robotic radiosurgery systems used thermoplastic masks only, but combined the masks with frequent image guidance (see Chapter 5) to ensure localization accuracy throughout treatment. The masks alone may yield an uncertainty of about 3 mm, but IGRT can reduce the uncertainty to the accuracy of the IGRT system. For example, the Accuray CyberKnife uses cross-firing x-ray cameras and automated robotic alignment to track and correct the position of the radiation beam throughout treatment (6).
EXTRACRANIAL IMMOBILIZATION
SRS treatments outside the cranium face additional challenges because of the nonrigid anatomy to be treated. The general requirements for stable, comfortable, and reproducible immobilization are compounded by the need to limit internal motion (both intra- and interfraction) and possible shifts in relative position between the target volume(s) and adjacent organs at risk. The use of IGRT in addition to immobilization is absolutely critical for stereotactic body radiation therapy (SBRT; see Chapter 5).
One of the original immobilization systems used in the development of SBRT was a stereotactic body frame developed at the Karolinska Institute (7), later sold by Elekta (Figure 4.4), which adopted several of the features of cranial stereotactic frames, including linear reference scales and fiducial markers for MR and CT imaging. Using this reference system, the coordinates of an internal target could be localized relative to the frame. The frame also included an abdominal compression plate to minimize respiratory motion during treatment. Marks on the patient at the chest and tibia were used to aid in reproducing patient position in the frame at CT and treatment. Using this frame, Blomgren et al. reported early experience of treating 31 patients for tumors in the lung, kidney, and liver (7).
FIGURE 4.4 Elekta body frame for stereotactic body radiosurgery.
Source: Image courtesy of Elekta.
Hamilton and Lulu (8) created an invasive body frame that could be attached to the vertebral spinal processes with the patient in a prone position for treatment of paraspinal lesions. The frame also included a stereotactic fiducial system that could be used to accurately localize the target volumes on the basis of CT imaging. Although the authors reported good localization accuracy (2 mm transverse and 4 mm longitudinal) with this frame, because of its invasive nature, it could be used only for single-fraction radiosurgery, and it was never widely adopted.
A noninvasive body frame for spinal SRS was developed at Memorial Sloan Kettering Cancer Center (MSKCC) (9), which created a more rigid immobilization of patients by incorporating both lateral and anterior pressure plates applied at bony anatomical points. Like the Karolinska frame, this system also incorporated fiducial markers and scales in the frame for stereotactic localization of body targets. The body frame was used in a special linear accelerator vault with a CT scanner. The patients would be transferred in the body frame from the CT couch to the linac couch for daily CT-based localization. Patient position was verified immediately before treatment with portal imaging. The authors reported approximately 3-mm reproducibility in the body frame between planning and treatment CT scans. Intratreatment motion average of 1.2 mm was reported, although this came from only two patients.
The BodyFix frame (Elekta) uses a double vacuum system, with a standard polystyrene sphere body cushion coupled with a cover sheet that is deflated over the patient, producing roughly uniform pressure to hold the patient into the frame during treatment (Figure 4.5). Li et al. (10) found that the device reduced intrafraction motion in patients receiving spine SBRT over vacuum cushion alone.
With the use of IGRT, even standard frames and masks used for conventional radiation therapy may be used for SBRT. However, special care should be taken to ensure the immobilization system is comfortable and stable throughout a long treatment. Positioning the patient with arms up may be advantageous for treatments in the thorax and abdomen, but may be very difficult for some patients to maintain. Positioning a lung cancer patient with the contralateral arm down may improve comfort and also beam clearance for some treatments.
50
FIGURE 4.5 BodyFix frame for stereotactic body radiosurgery. A double vacuum system is used to form a custom cushion under the patient and to hold the patient into the frame with a plastic sheet.
Source: Image courtesy of Elekta.
Radiosurgery targets in the head and neck and upper thorax or cervical spine are often set up using commercial thermoplastic mask systems. Mechalakos et al. (11), using kilovoltage imaging, reported interfraction setup errors of 2 to 3 mm, with intrafraction motion of less than 1 mm. The use of custom head cushions for each patient may reduce inter- and intrafraction motion (12).
MOTION MANAGEMENT
In conventional radiation therapy, large (1.5–2.0 cm) margins are typically placed around targets in the thorax and abdomen to account for respiratory-induced motion. These large margins might greatly increase the toxicity of high-dose SBRT treatments. Accordingly, methods of reducing respiratory-induced motion or restricting its effects have been incorporated in SBRT from the very beginning. These methods include abdominal compression, breath hold, and gated treatment delivery.
The first stereotactic body frame (13) by Lax et al. initially used a belt tightened to a fixed length to restrict abdominal motion. Using fluoroscopy, the authors noted a reduction in diaphragm motion from 1.5 to 2.5 cm without restriction to 0.5 to 1.0 cm with restriction. This same frame, commercialized by Elekta, later incorporated a compression plate that was connected to an arch over the patient via a screw. In simulation, fluoroscopy or four-dimensional (4D) imaging could be used to assess residual motion of the diaphragm under pressure, and adjustments could be made to restrict motion to a desired level, for example, to reduce target or diaphragm motion to less than 0.5 cm for treatment.
There are several disadvantages to this approach. The arch over the patient may reduce clearance of the gantry around the patient, particularly for noncoplanar beams. The pressure plate may be uncomfortable, particularly for elderly, frail patients. Also, the compression plate may create variable distortion of the anatomy beneath the plate during fractionated treatment (14). One method of producing moderate pressure has been reported, wherein the screw is tightened only until the operator observes the patient’s breathing switch from diaphragmatic to intercostal expansion (15).
More recent body frame designs, for example, the Body Pro-Lok frame from Civco, include options for both the rigid compression plate and a pneumatic pressure belt. The belt can be strapped around the patient at a fixed position and inflated to apply a fixed, uniform pressure across the abdomen. The belt system is commonly reported to be more comfortable than the plate. Another advantage of the belt is that the pressure is reported on a dial gauge. As the patient lies in the frame under pressure, he or she will often respond to the pressure by initially tightening the muscles in his or her abdomen. As time goes on, these muscles will relax, and this may slightly change the effective compression being applied. If the therapists observe a reduction in the pressure on the dial gauge during treatment, they may halt treatment, adjust the inflation of the belt to restore pressure, and resume.
Another approach to reducing the effects of respiratory motion is to use breath hold, either forced or voluntary, to minimize motion while the beam is on. Because of the very large fractional doses and high dose rates of stereotactic beams, breath hold, either forced or voluntary, must be carefully monitored throughout treatment, so that the beam may be terminated immediately if the patient cannot maintain breath hold. Breath hold may be done at either inspiration or expiration; patients are generally able to hold their breath longer at inspiration, and the greater inflation of the lungs at inspiration may reduce the volume of lung irradiated for lung SBRT targets. The two most common methods 51of breath hold are the spirometry-based active breathing coordinator (ABC) system (Elekta) and the video-based Real-Time Position Management (RPM) system (Varian).
The ABC system (16, 17) uses a mouth piece attached to a spirometer, with the patient’s nose plugged so that he or she can breathe only through the mouth piece. The spirometer is attached to a computer so that the operators can observe the breathing level. Once the patient breathes in to the required threshold, valves in the spirometer close, preventing the patient from breathing during treatment. A switch controlled by the patient will open the valve and allow him or her to breathe.
The Varian RPM system uses an infrared camera to monitor a marker block placed on the patient’s abdomen. The marker block is commonly placed near the xiphoid for breath-hold monitoring. For deep inspiration breath hold, the patient must voluntarily breathe in to the required level and hold his or her breath at that point. A visual signal is sometimes provided to the patient to assist him or her in breathing to the correct threshold. The RPM camera then records the position of the marker box, and the software can be set to trigger beam hold if the block moves beyond a certain set limit.
The breath-hold process generally requires compliant patients able to hold their breath for 15 to 20 seconds for the CT simulation process and for treatment. Because the breath hold is used for CT simulation, the CT images will be free of the motion artifacts commonly seen in planning scans of the chest and abdomen.
Finally, gating may be used to limit beam delivery to a fraction of the breathing cycle in which respiratory motion is minimal. For gated delivery, a detailed analysis of target motion, usually analyzed over a 4D CT scan (see Chapter 5), is performed. The operator then determines what fraction of the breathing cycle will limit target motion to a defined limit. For example, it may be determined that limiting the beam delivery to 50% of the breathing cycle, centered around end exhalation, will limit target motion to less than or equal to 0.5 cm. In general, the target motion is more reproducible and more stable for a longer period around end exhalation.
Gated treatment delivery does not require the patient to hold his or her breath or to be compressed in an uncomfortable frame; however, the method is predicated on the assumption that the breathing cycle of the patient is stable and reproducible and that the target motion tracks the motion of the external surrogate. Coaching via audio or visual signals may be used to improve the reproducibility of the patient’s breathing (18).
REFERENCES
1. Schell MC, Bova FJ, Larson DA, et al. Stereotactic radiosurgery. AAPM Report. 1995;54:30-36.
2. Sweeney R, Bale R, Vogele M, et al. Repositioning accuracy: comparison of a noninvasive head holder with thermoplastic mask for fractionated radiotherapy and a case report. Int J Radiat Oncol Biol Phys. 1998;41(2):475-483. PubMed PMID: 9607367
3. Ryken TC, Meeks SL, Pennington EC, et al. Initial clinical experience with frameless stereotactic radiosurgery: analysis of accuracy and feasibility. Int J Radiat Oncol Biol Phys. 2001;51(4):1152-1158. PubMed PMID: 11704340
4. Leksell L. The stereotaxic method and radiosurgery of the brain. Acta Chir Scand. 1951;102(4):316. PubMed PMID: 14914373.
5. Zeverino M, Jaccard M, Patin D, et al. Commissioning of the Leksell Gamma Knife® Icon™. Med Phys. 2017;44(2): 355-363. doi: 10.1002/mp.12052
6. Adler Jr JR, Chang SD, Murphy MJ, et al. The Cyberknife: a frameless robotic system for radiosurgery. Stereotact Funct Neurosurg. 1997;69(1-4):124-128. doi: 10.1159/000099863
7. Blomgren H, Lax I, Näslund I, et al. Stereotactic high dose fraction radiation therapy of extracranial tumors using an accelerator. Clinical experience of the first thirty-one patients. Acta Oncol. 1995;34:861-870. PubMed PMID: 7576756.
8. Hamilton AJ, Lulu BA. A prototype device for linear accelerator-based extracranial radiosurgery. Acta Neurochir Suppl. 1995;63:40-43. PubMed PMID: 7502726.
9. Yenice KM, Lovelock DM, Hunt MA, et al. CT image-guided intensity-modulated therapy for paraspinal tumors using stereotactic immobilization. Int J Radiat Oncol Biol Phys. 2003;55(3):583-593. PubMed PMID: 12573745.
10. Li W, Sahgal A, Foote M, et al. Impact of immobilization on intrafraction motion for spine stereotactic body radiotherapy using cone beam computed tomography. Int J Radiat Oncol Biol Phys. 2010;84(2):520-526. doi: 10.1016/j.ijrobp.2011.12.039
11. Mechalakos JG, Hunt MA, Lee NY, et al. Measurement of setup deviation in IMRT head and neck patients using an on-board kilovoltage imager. J Appl Clin Med Phys. 2007;8(4):2439. doi: 10.1120/jacmp.v8i4.2439
12. Courneyea L, Mullins J, Howard M, et al. Positioning reproducibility with and without rotational corrections for 2 head and neck immobilization systems. Pract Radiat Oncol. 2015;5(6): e575-e581. doi: 10.1016/j.prro.2015.05.003
13. Lax I, Blomgren H, Näslund I, et al. Stereotactic radiotherapy of malignancies in the abdomen. Methodological aspects. Acta Oncol. 1994;33(6):677-683. PubMed PMID: 7946448.
14. Eccles CL, Dawson LA, Moseley JL, et al. Interfraction liver shape variability and impact on GTV position during liver stereotactic radiotherapy using abdominal compression. Int J Radiat Oncol Biol Phys. 2011;80(3):938-946. doi: 10.1016/j.ijrobp.2010.08.003
15. Heinzerling JH, Anderson JF, Papiez L, et al. Four-dimensional computed tomography scan analysis of tumor and organ motion at varying levels of abdominal compression during stereotactic treatment of lung and liver. Int J Radiat Oncol Biol Phys. 2008;70(5):1571-1578. doi: 10.1016/j.ijrobp.2007.12.023
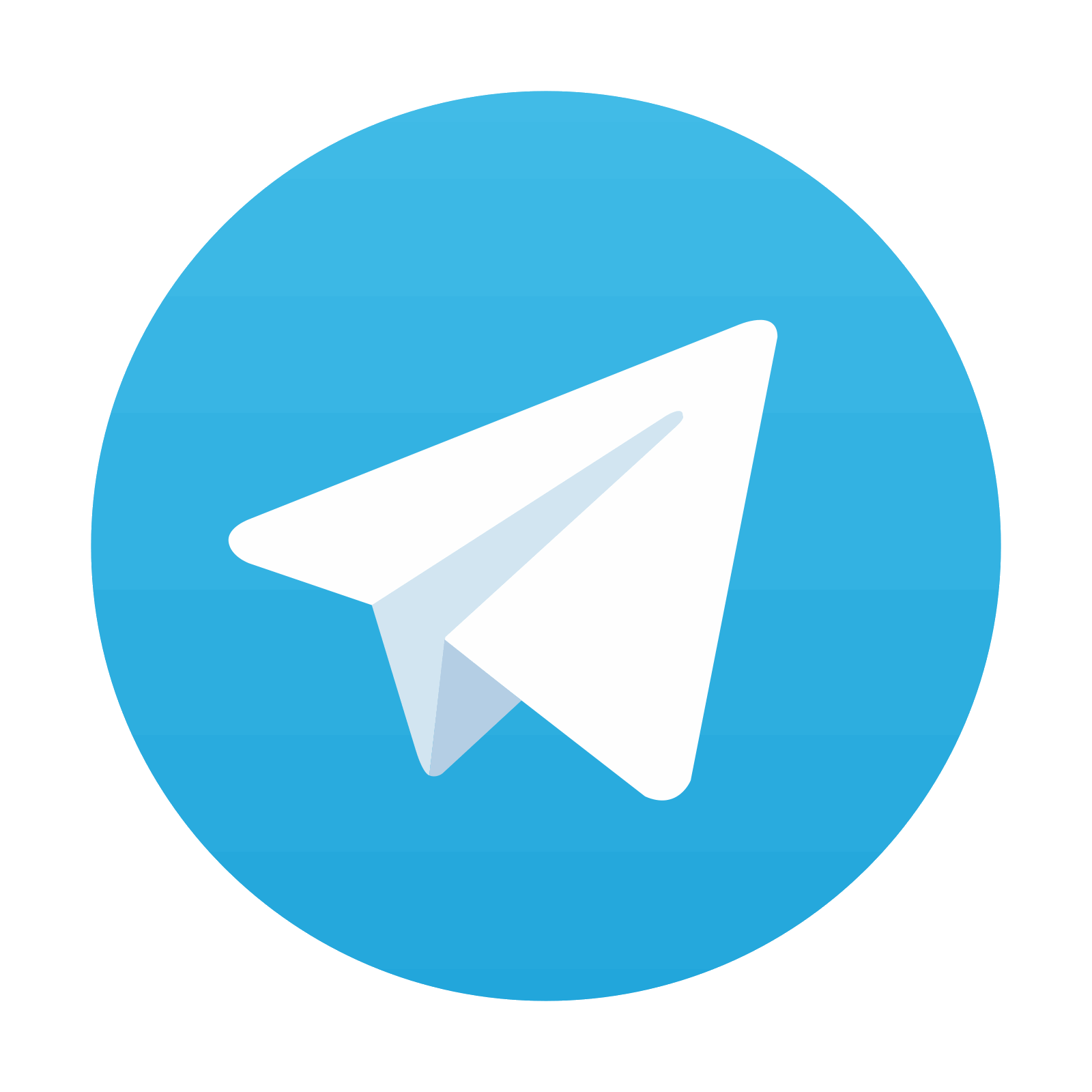
Stay updated, free articles. Join our Telegram channel
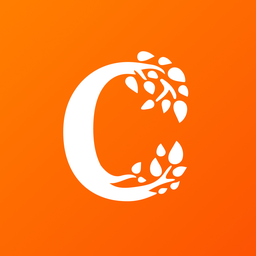
Full access? Get Clinical Tree
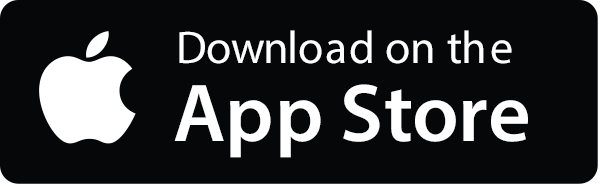
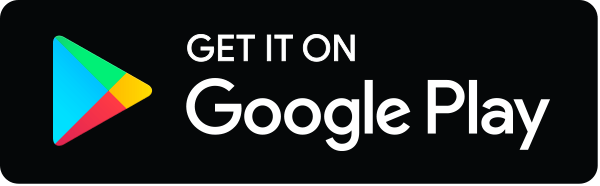