Thoracic MR imaging in the pediatric population provides unique challenges requiring tailored protocols and a practical approach to pediatric issues, such as patient motion and sedation. Concern regarding the use of ionizing radiation in the pediatric population has continued to advance the use of MR imaging despite these challenges. This article provides a practical approach to thoracic vascular MR imaging with special attention paid to pediatric-specific issues such as sedation. Thoracic vascular anatomy and pathology are discussed with an emphasis on protocols that can facilitate accurate diagnosis.
Key points
- •
Proper patient preparation is essential for thoracic MR imaging in pediatric patients.
- •
Sedation proves necessary to obtain diagnostic quality chest MR imaging in infants and young children.
- •
Various MR imaging techniques can visualize vascular anatomy both with and without the use of intravenous contrast.
- •
Evaluation of thoracic outlet syndrome is improved by dynamic MR imaging technique.
Introduction
Since its introduction to clinical practice, MR imaging has become a cornerstone of radiologic imaging. In recent years, advances in MR imaging technology including the use of 3T scanners and parallel imaging, have overcome many of the limitations that previously hindered the use of MR imaging for thoracic disorders. Particularly, the excellent soft tissue contrast and anatomic detail provided by MR imaging has encouraged its widespread adoption for thoracic vascular imaging. This review addresses practical strategies to improve thoracic MR image quality including overcoming motion artifacts and the necessity for sedation in many pediatric patients when performing thoracic vascular MR imaging. With tailored pediatric protocols, one can accurately diagnose myriad conditions without exposing the patient to ionizing radiation. Selected examples of pathology that vascular thoracic MR imaging can diagnose in the pediatric population are also provided.
Introduction
Since its introduction to clinical practice, MR imaging has become a cornerstone of radiologic imaging. In recent years, advances in MR imaging technology including the use of 3T scanners and parallel imaging, have overcome many of the limitations that previously hindered the use of MR imaging for thoracic disorders. Particularly, the excellent soft tissue contrast and anatomic detail provided by MR imaging has encouraged its widespread adoption for thoracic vascular imaging. This review addresses practical strategies to improve thoracic MR image quality including overcoming motion artifacts and the necessity for sedation in many pediatric patients when performing thoracic vascular MR imaging. With tailored pediatric protocols, one can accurately diagnose myriad conditions without exposing the patient to ionizing radiation. Selected examples of pathology that vascular thoracic MR imaging can diagnose in the pediatric population are also provided.
Technical issues: motion
Motion in chest imaging is typically caused by cardiac and respiratory activities. Cardiac gating relies on the use of an electrocardiogram (ECG) for monitoring the cardiac cycle during the MR imaging examination, allowing for the acquisition of images during diastole, the most quiescent portion of the cardiac cycle. Respiratory motion generally plays a much larger role in noncardiac thoracic imaging compared with cardiac motion. In an ideal setting, breath-hold imaging yields the highest quality images, as it can largely eliminate respiratory motion. Because the ability to perform breath holding is limited in infants and young children (<5 years old), this technique is reserved for older (≥5 years) or intubated children and, of course, requires rapid pulse sequences.
Another way to manage respiratory motion involves acquiring signal over a longer period, but only during the same phase of respiration. One can perform respiratory gating with the use of a bellows or belt strapped to the patient that monitors the respiratory cycle. Such methods can be deployed to selectively acquire MR signals only during end expiration, the portion of the cycle at which the abdomen and chest remain motionless. One can also monitor the phase of the respiratory cycle with the use a navigator echo. Navigator echoes allow either retrospective or prospective gating of signal by sampling a small area in the region of the diaphragm to assess diaphragmatic excursion. Similar to the use of the bellows, only signals acquired during the appropriate phase of the respiratory cycle are then used for final image reconstructions ( Fig. 1 ).
Children may have difficulty with extended breath holds or the concept of quiet breathing. In addition, the average resting respiratory rate increases with decreasing age. For this reason, multiple pulse sequences have been developed to optimize image quality. A simplistic way of categorizing these sequences is to divide them into sequences that only acquire data while the lungs are not moving and those sequences that acquire signal throughout the respiratory cycle in quiet breathing. Other methods to perform non–breath hold imaging involve faster sequences that undersample k space or sample k space in a fashion that minimizes motion artifact such as periodically rotated overlapping parallel lines with enhanced reconstruction (PROPELLER/BLADE).
Patient preparation issues: sedation
In the previous section, cardiac and respiratory motion were discussed as especially problematic to thoracic imaging. Even when optimizing the protocol using sequences such as PROPELLER that compensate for these two constant and implacable causes of motion, a moving patient can make a scan nondiagnostic. MR imaging relies on the acquisition of multiple series of images with the examinations taking anywhere between 15 minutes to more than an hour. Obtaining diagnostic MR imaging, therefore, requires either a cooperative or sedated patient.
The age of a patient plays a central role in developing an individualized imaging plan for each MR imaging examination. Older patients (typically older than 5 years) who can follow commands may attempt to undergo MR imaging without sedation. These pediatric patients, however, should undergo thorough preparation before the MR imaging examination is conducted either by the radiologist or medical staff familiar with the protocol to improve image acquisition. Children should be told what to expect with respect to the appearance of the magnet, the movement of the table, the loud noises they will hear, and the importance of laying still. Some institutions provide coaching in a mock scanner environment to improve familiarization with the noisy MR imaging environment in an attempt to further improve examination quality and success. Pediatric patients should also practice breathing instructions before the start of the examination. Although these steps result in additional time needed up front, they result in a high percentage of successful and diagnostic MR imaging examinations.
Some children, as in the adult population, may require sedation for MR imaging because of claustrophobia or other anxiety. Allowing the parent to stay in the room with the child, distraction methods such as goggles projecting a movie, or reassurance and coordination with ancillary staff accustomed to making children comfortable with imaging tests can often alleviate the need for sedation. Neonates can often undergo MR imaging without sedation by using the “feed and wrap” method wherein the child is fed immediately preceding the examination and then placed in the scanner after being swaddled. Although this technique works well in imaging of stationary body parts such as the brain, its application to chest imaging may prove limited given the sensitivity of MR imaging to respiratory motion and the high respiratory rates found in neonates, particularly those with underlying respiratory abnormalities.
Children younger than 5 and older children with cognitive delay or inability to follow commands require a different approach. A diagnostic MR imaging examination in this patient population mandates the use of deeper sedation, often with endotracheal intubation performed in conjunction with anesthesiologists. The risks of anesthesia should be weighed against the potential benefits of the imaging examination and should be explained to the parents of the patients. Recent studies in animal models have found that deep sedation in the early-developing brain may result in long-term sequelae. These patients in particular may benefit from another examination not requiring sedation, such as computed tomography (CT), if the risks to brain development from anesthesia outweigh the radiation risk.
Sedation of children in the radiology department has traditionally been performed with chloral hydrate because of its well-established efficacy and safety profile. Numerous other sedating agents have been used in children including fentanyl, midazolam, pentobarbital, propofol, chlorpromazine, and sevoflurane. Dexmedetomidine is a newer agent with a short half-life (approximately 2 hours) that is highly selective for the α2 adrenoceptor working at the level of the locus ceruleus in the central nervous system. This agent provides both sedation and analgesia with minimal effect on respiration. A recent study evaluating this agent in 279 patients within a radiology department found no adverse respiratory effects, no pharmacologic therapy needed to treat hemodynamic variability, and rapid recovery after the examination.
With the child sedated, the anesthesiologist can control patient respiration and potentially work in conjunction with the MR imaging technologist to time the acquisition of images. Hyperventilation, if properly timed, can recreate a breath hold type of state for those sequences requiring a breath hold. Alternatively, the anesthesiologist can hold respirations in a controlled environment with careful monitoring of the patient’s vital signs including pulse oximetry to obtain the images. The radiologist should minimize the amount of time the patient is sedated by ensuring that the provided imaging is diagnostic and not performing superfluous pulse sequences. In addition, MR imaging should be performed as quickly as possible once the patient is intubated to minimize the development of atelectasis, which can obscure underlying abnormality and can be secondary to patient ventilation. Once the examination is complete, the patient should be monitored in a controlled environment until the effects of anesthesia have worn off and the child is safe for discharge from the radiology department.
Angiography techniques
Traditional MR imaging has relied on the detection of precessing protons of the hydrogen atom in the body. Most soft tissues contain an abundance of water molecules, which, in turn, are excellent sources of protons and hence provide abundant signal. The vascular system also contains many circulating water molecules; however, their constant movement provides additional challenges and opportunities.
MR angiography provides a mechanism to evaluate the circulatory system in a less-invasive fashion than the traditional gold standard (ie, conventional angiography) and also provides unique advantages. Conventional angiography provides exquisite spatial and temporal resolution but can only image the patent lumen of the interrogated vessels. MR angiography, however, acquires images not only the lumen, but also the vessel wall, and surrounding structures. Although CT angiography provides much of the same data and is now considered standard of care for certain diagnoses such as pulmonary embolism, it requires exposing the patient to radiation and intravenous iodinated contrast.
One can perform MR angiography with and without contrast. Contrast-enhanced MR relies on the use of gadolinium to shorten T1 times and thereby increase the signal of the blood relative to the remainder of the body ( Fig. 2 ). This robust technique is less prone to flow-related image artifacts than the unenhanced sequences and provides excellent vascular imaging. The advent of 3T imaging improved image quality by increasing signal-to-noise ratio with shortened scan times and also allowing the use of lower gadolinium doses without degrading image quality. Depending on the timing of the image acquisition, traditional gadolinium contrast may be found primarily in the arterial system or venous system or diluted throughout both. The use of newer intravascular gadolinium agents, such as gadofosveset, facilitates vascular studies by keeping the contrast within the blood vessels allowing exquisite vascular delineation for an extended period without significant signal loss. If only a certain portion of the vasculature (ie, arterial system) is of interest, selective scanning early during the contrast injection cycle allows for delineation between the opacified signal-rich arteries and the signal-poor veins.
In some situations, current clinical guidelines preclude the use of gadolinium. Patients with a glomerular filtration rate of less than 30 mL/min are at risk of nephrogenic systemic fibrosis, a scleroderm alike condition. Although certain formulations of gadolinium are considered low risk for development of nephrogenic systemic fibrosis because of improved stability between the chelate and gadolinium, a glomerular filtration rate less than 30 mL/min generally precludes the use of contrast in our institution. Administering contrast in this situation would require a careful analysis of the risk-benefit ratio, informed consent from the parents, and nephrology consultation. The guidelines have also changed regarding the use gadolinium in children less than 1 year of age. In this population, gadolinium is contraindicated in neonates less than 4 weeks old and should be used cautiously in patients less than 1 year old. In pregnant patients, the use of gadolinium is often generally avoided because of the theoretic, but unproven, risk to the fetus unless the benefits outweigh the potential risk. In all these situations, non–contrast-enhanced MR angiography provides an excellent alternative. An added benefit of non–contrast-enhanced MR angiography is the ability to perform multiple sequential acquisitions should the initial images prove nondiagnostic.
Time-of-flight MR angiography relies on the use of either 2-dimensional or 3-dimensional gradient echo sequences in which the constant inflow of blood into the imaging slab results in a higher signal intensity within the vessel than the surrounding soft tissues saturated by repeated radiofrequency pulses. These sequences are limited by long acquisition times and signal loss when blood flow is parallel to the imaging plane. Consequently, time-of-flight MR angiography can underestimate blood flow. Additional causes of signal loss include turbulent flow and susceptibility artifact associated with gradient-echo imaging.
Steady-state free precession images (SSFP), with a complicated T1 and T2 weighting, can provide anatomic information with good spatial and temporal resolution. These properties have made SSFP one of the main sequences of cardiac imaging. Numerous studies have found that SSFP sequences accurately diagnose pathology in the aorta and smaller vessels. SSFP sequences have high signal-to-noise ratio and are relatively independent of flow directionality; however, the sequences are susceptible to field inhomogeneities, especially at higher field strengths. The use of arterial spin labeling together with SSFP sequences has also been applied to angiographic imaging; however, discussion of this topic is beyond the scope of this article.
Double-inversion recovery imaging suppresses signal from flowing blood resulting in a black blood image. By eliminating signal from the flowing blood, one can better evaluate mural abnormalities. Black blood imaging requires ECG gating, resulting in long acquisition times. The use of different k space reconstruction algorithms (PROPELLER) is useful in decreasing motion artifact even in the absence of gating.
ECG-gated fast spin echo MR angiography provides bright blood vascular imaging by subtracting systolic images from diastolic images. In systole, the fast blood flow results in a signal void. During diastole, the more slowly flowing blood retains its signal. Consequently, the subtraction images eliminate the signal from the background tissues and only retain the fast flowing blood. Limitations of this technique include the need for appropriate ECG triggering and sensitivity to motion, especially as subtraction of images is involved.
MR imaging is an excellent imaging modality for evaluation of thoracic vasculature. Routine MR imaging can often detect congenital aortic arch abnormalities such as a right-sided or double aortic arch. A fundamental protocol would begin with a core number of T1-weighted and T2-weighted sequences. Additional angiographic pulse sequences as described above provide additional information about the blood vessels. The use of contrast-enhanced MR angiography sequences with gadolinium increases the accuracy for detection of extracardiac mediastinal vascular abnormalities; however, the decision to administer contrast requires a careful weighing of both clinical necessity and risk factors. Newer blood pool contrast agents such as gadofosveset (Ablavar) continue to improve vascular imaging and may further increase diagnostic accuracy.
Evaluation of extracardiac mediastinal vascular structures
Vascular Rings and Slings
MR imaging can optimally illustrate congenital mediastinal vascular variants. Pediatric patients with unexplained dysphagia or respiratory symptoms often undergo evaluation for the presence of vascular rings, most commonly a double aortic arch or a right-sided aortic arch with an aberrant left subclavian artery. Although the diagnosis is often suggested by the impressions on the esophagus best seen on fluoroscopic contrast studies, confirmation and preoperative assessment typically rely on cross-sectional imaging ( Fig. 3 ). In the case of the double aortic arch, it is important for the surgeon to know which arch is dominant, as the contralateral arch is typically ligated, and such information affects the surgical approach ( Fig. 4 ). A left-sided aortic arch with an aberrant right subclavian is the most common arch anomaly, occurring in up to 2.5% of the general population; however, this congenital vascular anomaly does not represent a true vascular ring ( Fig. 5 ). This variant is typically asymptomatic; however, in some cases it results in dysphagia lusoria or coughing. Operative management, although controversial, has been used in symptomatic cases refractory to conservative management.
Another mediastinal vascular variant that MR imaging can optimally illustrate is a pulmonary artery sling, in which the left pulmonary artery arises from the right pulmonary artery and passes posterior to the trachea and anterior to the esophagus ( Fig. 6 ). Although the characteristic impressions on the trachea and esophagus can be seen on a lateral radiograph or fluoroscopic study, MR imaging or CT permit direct visualization.
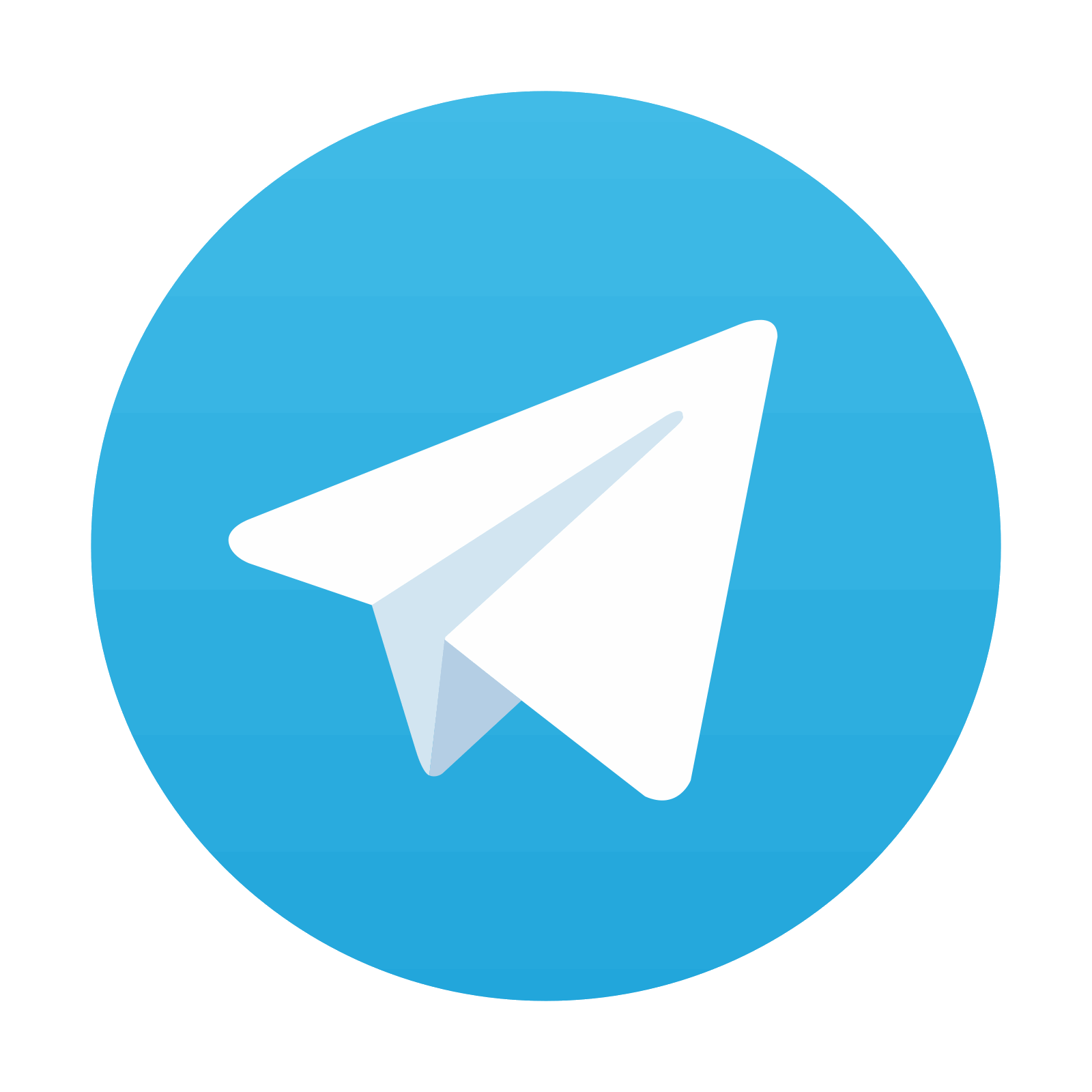
Stay updated, free articles. Join our Telegram channel
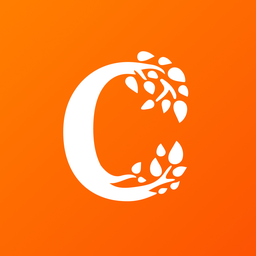
Full access? Get Clinical Tree
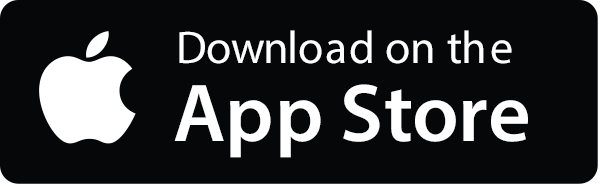
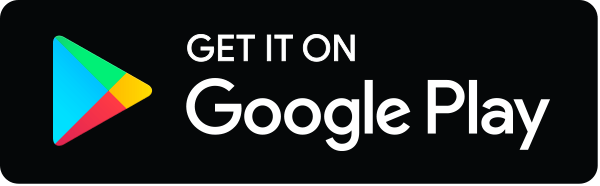
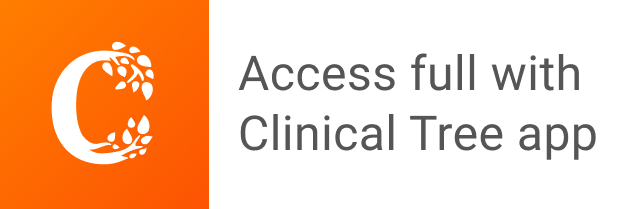