Type of dystonia
Prominent symptoms
Etiology
Focal
Cervical
Dystonia of the neck muscles
Unknown
Blepharospasm
Dystonia of the eyelids
Unknown
Writer’s cramp
Task-specific dystonia of the hands
Unknown
Focal hand dystonia
Non-task-specific dystonia of the hands
Unknown
Oromandibular dystonia
Dystonia of the jaw and tongue
Unknown
Spasmodic dysphonia
Dystonia of the vocal cords
Unknown
Segmental dystonia
e.g., Meige syndrome
Blepharospasm with dystonia of the jaw or lower face muscles
Unknown
Multifocal dystonia
Hemidystonia
e.g., poststroke dystonia
Dystonia of the arm and leg on one side of the body
Usually poststroke
Generalized dystonia
Nongenetic
Generalized dystonia
Usually post-anoxia, e.g., birth trauma
DYT1
Generalized dystonia starting in the limbs
TorsinA gene mutation
DYT6
Focal or generalized dystonia starting in the cranial or cervical muscles
THAP1 gene mutation
Dystonia-plus syndromes
Dopamine-responsive dystonia
Generalized dystonia starting in one leg that responds to levodopa
GCH1 gene or SRG gene mutation
Myoclonus-dystonia
Myoclonus, dystonia, and psychiatric symptoms
SGCE gene mutation
Rapid-onset dystonia-parkinsonism
Acute-onset dystonia and parkinsonism
ATP1A3 gene mutation
Paroxysmal dystonia
Paroxysmal kinesigenic dystonia (DYT9 and DYT10)
Attacks of generalized dystonia triggered by sudden movements
DYT9 = unknown gene mutation on locus 1p21-p13.3
DYT10 = PRRT2 gene mutation
Paroxysmal non-kinesigenic dystonia (DYT8 and DYT20)
Spontaneous attacks of generalized dystonia
DYT8 = PNKD1 gene mutation
DYT20 = unknown gene mutation on locus 2p31
Paroxysmal exercise-induced dystonia (DYT18)
Attacks of generalized dystonia triggered by prolonged exercise
GLUT1 gene mutation
Primary focal dystonia is dystonia of unknown origin in one body part. The most common form of focal dystonia is CD. Other forms are, for example, blepharospasm (BLS, dystonia of the eyelids), writer’s cramp (WC) or focal hand dystonia, oromandibular dystonia (dystonia of the jaw), and spasmodic dysphonia (dystonia of the vocal cords). WC tends to debut around the age of 40 years, CD around the age of 50 years, and BLS around 60 years. One of the most extraordinary features of focal dystonia is that it can be task specific, a feature that is often used in imaging studies. The most well-known form of task-specific dystonia is WC where patients get a dystonic posture of the hand while trying to write, but not in rest or during other hand movements (Phukan et al. 2011).
Patients with generalized dystonia usually develop dystonia in childhood or during adolescence. These patients frequently have a genetic cause for dystonia, and most of these familial syndromes have been allocated a DYT number based on clinical characteristics. The DYT syndromes have been numbered consecutively in order of the discovery of the clinical syndrome. For several DYT numbers, the causative gene has been identified (Muller 2009). Two forms of generalized dystonia that have been well studied are DYT1 and DYT6 dystonia. Onset of dystonia in DYT1 is usually in childhood, and the first symptoms are typically in the limbs. Dystonia generalizes over the course of a few years in most cases. DYT1 dystonia is caused by a mutation in the TorsinA gene. The penetrance of the mutation is 30 %, meaning that only 30 % of gene carriers develop dystonia during their lifetime (Spatola and Wider 2012).
DYT6 is the second most common genetic cause of hereditary dystonia. Age at onset is more variable than in DYT1 dystonia, varying from onset in childhood to adult onset. Dystonia typically starts in cranial or cervical muscles after which it slowly progresses. Dysphonia is a common symptom in DYT6 dystonia. DYT6 dystonia is caused by a mutation in the THAP1 gene. This mutation also has reduced penetrance: 60 % of gene carriers develop dystonia (Spatola and Wider 2012).
Dopamine-responsive dystonia (DRD, also known as Segawa syndrome), has been named DYT5 dystonia. DRD usually starts in childhood with dystonia of a leg. After this, dystonia usually progresses and patients also develop parkinsonian features (Muller 2009) (Asmus and Gasser 2010). DRD is most commonly (~80 % of cases) caused by a mutation in the GCH1 gene encoding GTP-cyclohydrolase-1, an enzyme necessary for the production of levodopa and dopamine. This mutation is transmitted in an autosomal dominant fashion with a penetrance of ~30 % (Asmus and Gasser 2010; Muller 2009). Mutations have also been found in the sepiapterin reductase gene (SRG) and the tyrosine hydroxylase (TH) gene. Both these enzymes are also involved in the production of dopamine (Gordon 2008; Hoffmann et al. 2003). All known mutations lead to reduced production of endogenous dopamine (Asmus and Gasser 2010; Muller 2009).
Myoclonus-dystonia (MD, DYT11) is also one of the dystonia-plus syndromes. Onset is usually in childhood or adolescence with myoclonic jerks, later followed by a relatively mild form of dystonia. MD is caused by a mutation in the epsilon-sarcoglycan gene (SGCE gene) in 30–40 % of all cases and is transmitted in an autosomal dominant matter with reduced penetrance and maternal imprinting (Kinugawa et al. 2009). The latter means that only gene carriers inheriting the gene from their father can get the disease. This is largely true, but a small percentage of patients inheriting the gene from their mother also have (mild) symptoms (Kinugawa et al. 2009). In most SGCE-negative cases, no genetic defect can be identified although a novel locus on chromosome region 18p11 has been identified. The causative gene in this locus is unknown (Grimes et al. 2002).
Rapid-onset dystonia-parkinsonism (RDP, DYT12) is an autosomal dominant disease with abrupt onset of dystonia and parkinsonism over days to weeks, followed by little or no improvement. Permanent and disabling dystonia presents acutely after physical or psychological stress, fever, or alcohol excess. RDP is caused by mutations in the α3 subunit of the Na/K-ATPase, the ATP1A3 gene (Schneider and Bhatia 2010).
Paroxysmal dystonia is a group of disorders (DYT8, DYT9, DYT10, DYT18, and DYT20) that is characterized by attacks of dystonic movements and postures with sometimes chorea or myoclonus. The disorders that can be distinguished are paroxysmal kinesigenic dystonia (PKD, attacks of several seconds triggered by sudden movements), paroxysmal non-kinesigenic dystonia (PNKD, spontaneous attacks lasting minutes to several hours), and paroxysmal exercise-induced dystonia (PED) (van Rootselaar et al. 2009). The most common form, PKD, was recently shown to be caused by mutations in the PRRT2 gene (Wang et al. 2011). Exercise-induced dystonia has recently been found to be caused by a mutation in the GLUT1gene, encoding a glucose transporter in the blood–brain barrier. Due to the mutation in this gene, there is reduced glucose transport to the brain (Friedman et al. 2006).
31.1.4 Pathophysiology
The pathophysiology of dystonia is still largely unknown even for the genetic forms of dystonia. It is unclear what the function of the TorsinA, THAP1, GCH, SRG, and SGCE genes is and what happens at a cellular level when these genes are mutated. Sporadic forms of dystonia are thought to be caused by a combination of genetic and environmental factors (Tarsy and Simon 2006).
Dystonia has been hypothesized to be a so-called network disorder. Evidence for this network model has been gathered over the years in studies on secondary dystonia, in animal models, and in imaging studies, mainly resting-state fMRI and resting-state FDG-PET (Hendrix and Vitek 2012; Poston and Eidelberg 2012). The basal ganglia have been implicated in the pathophysiology of dystonia since the first cases of secondary dystonia were described with a lesion in the basal ganglia and/or thalamus (Kostic et al. 1996; LeDoux and Brady 2003; Lee et al. 1994). Later functional abnormalities were also found in the mean discharge rates of the globus pallidus externa (GPe) and the globus pallidus interna (GPi) using microrecordings. Patients with dystonia showed lower mean discharge rates compared to controls. This was hypothesized to lead to a disruption of the function of the basal ganglia-thalamocortical motor circuit and would therefore affect the ability to properly focus movements (Vitek et al. 1999). This hypothesis was confirmed in an animal model in which neurons in the mouse equivalent of the GPi were found to show irregular burst activity during dystonic posturing. Spontaneous remission of the dystonic behavior was seen at the same time as normalization of the neuronal discharge pattern (Gernert et al. 2002).
Dopamine, one of the main neurotransmitters in the basal ganglia, is believed to play an important role in the pathophysiology of all forms of dystonia and has been one of the points of focus in PET and SPECT imaging. One argument for the role of dopamine is DRD, which can successfully be treated with levodopa (Naumann et al. 1997). Other important arguments are the coexistence of dystonia in patients with Parkinson’s disease, especially in patients that develop PD at a young age and patients that have been treated with levodopa for longer periods (Wickremaratchi et al. 2009), and the possibility of developing dystonia after use of antipsychotics (dopamine D2/3R blockers) (Haddad and Dursun 2008).
Besides the basal ganglia, the sensory system has also been implicated in the pathophysiology of dystonia. One of the main arguments for disturbed sensory processing was expanded digit representation and abnormal overlap of digit representation in animals (Byl et al. 1996, 1997, Byl 2004) and patients with focal hand dystonia (Sanger et al. 2001; Tempel and Perlmutter 1990; Tinazzi et al. 2000). Altered sensory processing has been found not only in the sensory cortex but also in the basal ganglia in dystonia, suggesting that dystonia may also partly be a sensory disorder (Hendrix and Vitek 2012).
The role of the cerebellum and brain stem in the pathophysiology of dystonia has also been of interest. Studies in cat have showed that stimulation of the cerebellar nuclei alters the firing rates and dopamine levels in the basal ganglia (Li and Parker 1969; Nieoullon et al. 1978; Ratcheson and Li 1969). The role of the cerebellum and brain stem has been affirmed by case reports of patients with secondary dystonia after a lesion in one of these areas (Kostic et al. 1996; LeDoux and Brady 2003).
FDG-PET studies have helped to bundle the findings from these different brain areas into a network model by identifying two patterns: a torsion dystonia-related pattern (TDRP) which is affected in patients with sporadic dystonia and genetic dystonia, as well as in non-manifesting gene carriers (Eidelberg et al. 1995; Eidelberg 1998; Hutchinson et al. 2000; Trost et al. 2002), and a dystonic symptom-specific pattern only affected in DYT1 and DYT6 patients, but not in non-symptomatic gene carriers (Carbon and Eidelberg 2009) (also see the section on hereditary generalized dystonia).
In summary, the evidence from all these brain areas implicate the cortico-striato-pallidothalamocortical and cerebello-thalamocortical circuits in the pathophysiology of dystonia and support the hypothesis of dystonia as a network disorder.
31.1.5 Treatment
Treatment differs for the different types of dystonia. Most forms of focal dystonia are treated locally with intramuscular botulinum toxin (BTX) injections (Zoons et al. 2012). BTX can also be used in patients with generalized dystonia or MD in the body part that is most affected or causes most disability. Usually patients with generalized dystonia are treated with drugs, e.g., trihexyphenidyl (Artane), benzodiazepines, or baclofen, or with deep brain stimulation (DBS). Patients with DRD are treated with small amounts of levodopa with dramatic treatment response. The treatment for paroxysmal dystonia differs according to the nature of the syndrome and can consist especially of antiepileptics (e.g., carbamazepine) for PKD and a ketogenic diet (mainly in PED) (Muller 2009; Tarsy and Simon 2006).
31.2 Imaging with PET and SPECT in Different Forms of Dystonia
PET and SPECT studies have been performed in patients with different forms of dystonia. The studies that have been performed can roughly be divided in three types: PET studies for glucose metabolism, PET studies for regional cerebral blood flow (rCBF activation studies), and PET and SPECT studies for receptor imaging, mainly dopamine, but also the serotonin, GABA, and opioid systems have been studied. The information in this chapter is divided by form of dystonia, starting with focal dystonia followed by generalized dystonia, and dystonia-plus syndromes: DRD, MD, RDP, and paroxysmal dystonia. We will not discuss the results in secondary dystonia. As mentioned above, the causes for secondary dystonia are numerous, and the abnormalities found with imaging are usually a result of the underlying condition and not of dystonia per se.
31.3 Primary Focal Dystonia
Several PET and SPECT studies have been performed over the years trying to unravel the pathophysiology of focal dystonia (for a recent review see Zoons et al. 2011).
31.3.1 Glucose Metabolism PET
Using [18F]-fluorodeoxyglucose (FDG) PET, increases and decreases in FDG uptake have been found in the basal ganglia, cerebellum, and sensorimotor cortex of patients with focal dystonia. A bilateral increase in glucose metabolism in the basal ganglia, thalamus, lentiform nucleus, premotor-motor cortex, and cerebellum has been found in patients with CD compared to controls (Galardi et al. 1996; Magyar-Lehmann et al. 1997). There was an increase in glucose metabolism in the striatum and thalamus of patients with BLS and Meige syndrome (combined dystonia of the eyes and mouth/jaw) compared to controls (Esmaeli-Gutstein et al. 1999) and in the cerebellum and pons in patients with BLS compared to controls (Hutchinson et al. 2000). During induced sleep, patients with BLS showed glucose hypometabolism in the superior-medial aspect of Brodmann area 8 compared to controls. This region is associated with supranuclear control of eyelid opening (Hutchinson et al. 2000). The glucose hypermetabolism in the cerebellum and the pons has been replicated in a study that examined patients with BLS after treatment with BTX. A bilateral glucose hypermetabolism in the thalamus and the pons of patients with BLS was found compared to controls as well as a trend toward glucose hypermetabolism in the putamen bilaterally. Patients with incomplete suppression of BLS on BTX had glucose hypermetabolism in the cerebellum and the pons compared to patients with complete suppression (Suzuki et al. 2007). Furthermore, a complex network of cortical and subcortical regions with increased and decreased metabolism was found in patients with BLS compared to controls. There was increased metabolism in the inferior frontal gyri (Brodmann areas 45 and 46 bilaterally), right posterior cingulate gyrus, left middle occipital gyrus, fusiform gyrus of the right temporal lobe, left anterior cingulate gyrus, and caudate nucleus. Decreased glucose metabolism was found in the inferior frontal gyri (Brodmann area 47 bilaterally), left cerebellar hemisphere, and thalamus. Lateralization of activation of the frontal and temporal cortex to the right with contralateral deactivation of the left cerebellum suggests a connection mediated by the corticopontocerebellar pathway (Kerrison et al. 2003).
In summary, both patients with CD and BLS showed hypermetabolism in the basal ganglia, thalamus, and cerebellum in most studies, although cerebellar hypometabolism has also been described in patients with BLS. A network of other cortical and subcortical areas could also be affected in patients with BLS.
31.3.2 Regional Cerebral Blood Flow Activation PET
Where the above-described studies using [18F]-FDG found abnormalities in glucose metabolism mainly in the basal ganglia, the abnormalities in regional cerebral blood flow activation studies (rCBF activation studies) as measured with [15O]-H2O are mainly cortically localized. Similar to fMRI, such distribution reflects subtle changes within extended circuitry including outflow targets of basal ganglia – thalamic loops. [15O]-H2O PET is often used in task-related studies on WC patients. Due to the short half-life of [15O] (2 min), repeated studies can be performed in a short time period (Mishina 2008).
One study showed impaired activation of the primary motor cortex and greater activation in frontal and parietal association areas in WC patients compared to controls while writing. BTX treatment failed to normalize the impaired activation of primary motor cortex but did enhance activation of parietal cortex and accessory motor areas (Ceballos-Baumann et al. 1997). Patients with WC also showed reduced rCBF activation in sensorimotor and premotor structures in different tasks compared to controls. Certain regions were significantly abnormal for specific tasks. Patients showed significantly less rCBF activation in the contralateral vs. ipsilateral primary sensorimotor cortex, during sustained flexion or extension of the wrist. There was a significant decrease of rCBF activation in the left premotor cortex with writing, but there were no differences during tapping (Ibanez et al. 1999). A significant increase was found in rCBF activation of the primary sensory cortex and decrease of rCBF activation of the supplementary motor area (SMA) in patients with WC during writing and tapping compared to controls. Increased activation of the primary sensory cortex found in this study might reflect more intense processing of the sensory information or possibly expanded cortical representation of the hand area. The investigators also found increased activation of the right cerebellum of patients with WC compared to controls (Lerner et al. 2004). In patients with CD using a sensory trick, a significant decrease of motor cortical activation contralateral to the side toward which the head tends to rotate was found. This modulation includes the SMA (anterior part), the part of the precentral gyrus, and the primary sensorimotor cortex (SMC). In addition, the sensory trick leads to an increased activation of the parietal cortex ipsilateral to the direction of dystonic head rotation and bilateral occipital cortex (Naumann et al. 2000). A study of patients with facial dystonia (blepharospasm and oromandibular dystonia) showed a significantly reduced primary sensorimotor area (PSA) activation response to vibration of the lower face in patients with facial dystonia compared to healthy controls. The peak activations the authors observed in this study were centered in the precentral gyrus, adjacent to the central sulcus, consistent with the primary motor cortex (Feiwell et al. 1999). The only study using [15O]-butanol in patients with WC found increased blood flow in the contralateral thalamus and primary sensorimotor and premotor cortical areas but also in the ipsilateral cerebellum as the patients with WC invoked progressively greater dysfunction by a longer duration of writing (Odergren et al. 1998).
In conclusion, abnormalities in rCBF activation showed larger variety in results than those in glucose metabolism. This is intrinsically related to the difference between task-evoked and resting-state patterns in rCBF and metabolic PET measurements, respectively. Other differences between the studies could have been caused by a number of factors. Most studies evaluated patients with WC, but patients with CD, BLS, and oromandibular dystonia were also included, which could have attributed to different results. Indeed, more importantly most rCBF activation PET studies used tasks during the scans. Some tasks, especially a writing task in patients with WC, could have lead to dystonic symptoms, while other tasks, e.g., tapping, did not. In a recent review it was shown that in fMRI studies tasks that induced dystonia in general lead to an increase in activation in the cortical motor areas, basal ganglia, and cerebellum, while tasks that do not induce dystonia lead to a decrease in these same areas (Zoons et al. 2011). This could also explain the differences in the above-described rCBF activation PET studies. In the above-described rCBF activation PET studies that compared writing with rest or tapping, decreased rCBF activation was found in the PMC, premotor areas, and SMA, consistent with results from fMRI studies. As mentioned, rCBF activation PET is mainly used to quantify relative rCBF changes during tasks, thus enabling a comparison of brain activity between tasks, between tasks and rest, and between groups. In contract to rCBF activation studies, glucose metabolism PET is performed during rest and reflects a more long-term brain activity (due to the longer half-life of the tracer). In this way, the results from these two techniques complement each other.
31.3.3 Receptor Imaging with PET and SPECT
Two PET studies looked at D2/3 receptor (D2/3R) availability in patients with focal dystonia. Leenders and co-workers found no difference in striatal [11C]-N-methyl-spiperone (NMSP) binding to D2-like receptors in patients with mainly CD when compared to healthy controls. A trend was observed regarding the side-to-side differences: specific binding of the tracer tended to be higher in the striatum contralateral to the side to which the head was turning to (Leenders et al. 1993). Another study showed decreased [18F]-spiperone (SP, another D2-like receptor tracer) binding in the putamen of patients with hand and facial dystonia. There was no significant difference between patients with hand and facial dystonia (Perlmutter et al. 1997).
In patients with CD the average specific striatal binding to D2/3Rs, measured with [123I]-iodobenzamide ([123I]-IBZM) SPECT, was not significantly different from the control group. However, patients exhibited a more asymmetric striatal binding compared to controls, and 50 % of the patient group did show a higher receptor binding in the striatum contralateral to the direction of head rotation. The difference in binding was statistically significant for this patient group (Hierholzer et al. 1994). This is in accordance with the increased dopamine receptor binding in the contralateral striatum found in the PET study in patients with CD mentioned above and has also been found in other SPECT studies (Leenders et al. 1993). For example, a tendency for a reduced IBZM binding in the dorsal portion and an increase in the ventral parts of the striatum contralateral to the side of head rotation was found by Becker et al. in CD patients, but these results did not reach statistical significance, probably because of small study size (n = 10) (Becker et al. 1997). The low IBZM binding contralateral to the side of head deviation may indicate a reduced postsynaptic D2/3R density within the lentiform nucleus. These findings may point toward the medial lentiform nucleus or pallidothalamic pathway as the site of pathology in CD (Becker et al. 1997). One study evaluated both the pre- and postsynaptic dopaminergic system in patients with CD, using [123I]-epidepride (a tracer for D2/3R) and [123I]-β-CIT (a tracer for the presynaptic dopamine transporter = DAT). Striatal D2/3R binding was bilaterally significantly reduced in patients compared to controls, but there was no difference in striatal DAT binding (Naumann et al. 1998). In a study evaluating patients with WC, the striatal D2/3R binding was bilaterally decreased in patients compared to controls (Horstink et al. 1997). Another SPECT study evaluated patients with WC pre- and post-biofeedback-based sensorimotor training with [123I]-IBZM SPECT and also found decreased striatal binding. The training made patients more confident to write with a relaxed limb, writing improved, and striatal D2R binding restored to nearly normal levels (Berger et al. 2007). One study evaluated the integrity of cholinergic nerve terminals in patients with CD using [123I]-iodobenzovesamicol ([123I]-IBVM), a SPECT tracer that binds to the vesicular acetylcholine transporter (VAChT). They found a reduced IBVM binding in the putamen as well as in the whole striatum in patients with CD compared to controls (Albin et al. 2003).
In summary, the findings in PET and SPECT studies implicate a role for the striatal dopaminergic system and the basal ganglia in the pathophysiology of focal dystonia.
31.3.4 Similarities Between Different Forms of Focal Dystonia
Whether different forms of primary focal dystonia share an underlying pathophysiological mechanism remains a matter of debate. Some of the found abnormalities are strikingly similar, although there are also differences between the imaging findings in different forms of focal dystonia. In both CD and BLS, increased glucose metabolism was found in the basal ganglia, thalamus, and cerebellum. Brodmann area 8 (supranuclear control of eyelid opening) was only found to be hypoactive in patients with BLS and could underlie a symptom-specific abnormality. A network of areas with increased and decreased metabolism has only been found in BLS, but has also not been investigated in other forms of focal dystonia. Studies using rCBF activation PET are more difficult to compare between the different forms of focal dystonia since most studies in patients with WC used tasks, while studies in patients with CD and BLS did not. In patients with WC the PMC shows decreased rCBF activation, as it does in patients with BLS during facial vibration. It is unclear how these findings relate to each other. Receptor imaging has showed decreased D2/3R binding in CD, BLS, and WC, indicating a hyperdopaminergic state might be part of the pathophysiology of all forms of focal dystonia.
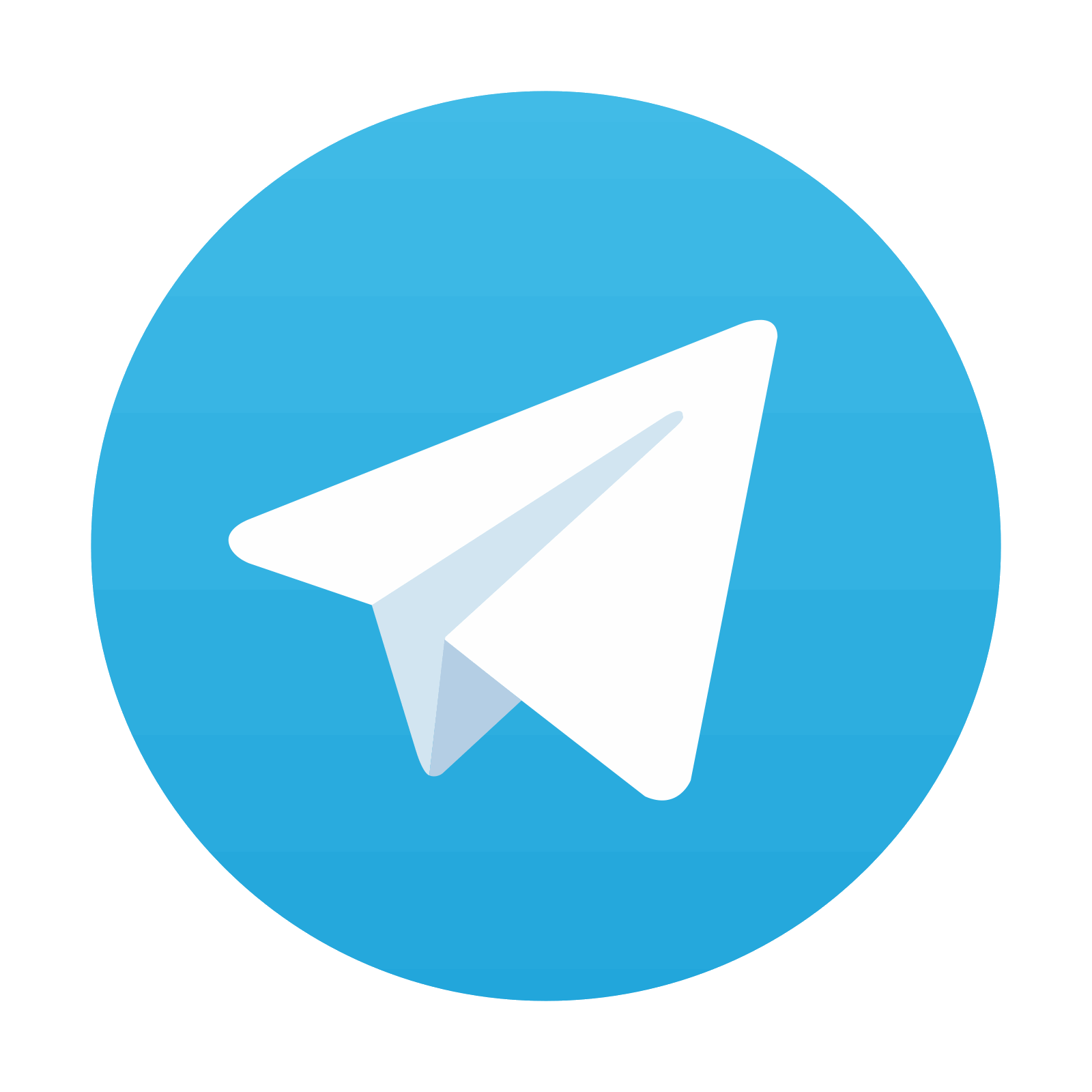
Stay updated, free articles. Join our Telegram channel
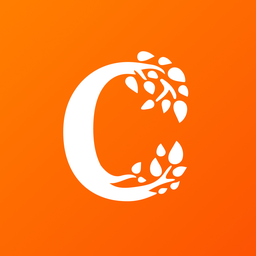
Full access? Get Clinical Tree
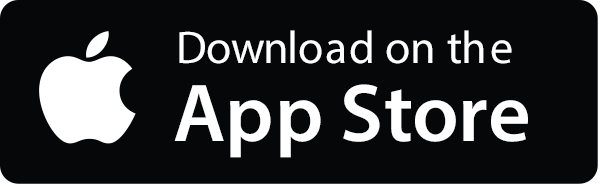
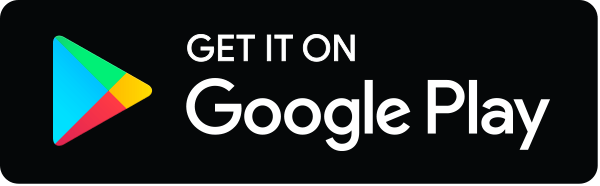