Physical Principles and Bioeffects of First Trimester Ultrasound
INTRODUCTION
Recent advances in ultrasound technology along with a growing body of literature expanded the role of obstetric ultrasound in the first trimester. Currently, first trimester ultrasound is considered an important element of pregnancy care and is clinically used to accurately date a pregnancy, assess for risk of aneuploidy, and screen for major fetal malformations. Understanding the basic physical principles of ultrasound is essential for knowledge of instrument control and also for the safety and bioeffects of this technology. In this chapter, we present the basic concepts of the physical principles of ultrasound, define important terminology, and review its safety and bioeffects, especially with regard to its use in the first trimester of pregnancy. Following chapters will present the role of first trimester ultrasound in pregnancy dating and in screening for fetal malformations.
PHYSICAL CHARACTERISTICS OF SOUND
Sound is a mechanical wave that travels in a medium in a longitudinal and straight-line fashion by transmitting its energy from one molecule to another. Sound therefore cannot travel in vacuum as it requires a medium for energy transfer. When sound travels through a medium, the molecules of that medium are alternately compressed (squeezed) and rarefied (stretched). It is important to note that the molecules oscillate but do not move as the sound wave passes through them. Seven acoustic parameters describe the characteristics of a sound wave and are listed in Table 2.1. In this chapter, we will briefly discuss the frequency, power, and intensity of sound given their importance to safety of ultrasound. For more details and a broader discussion on ultrasound physics, the readers are directed to references on this subject.1, 2, 3
The frequency of a sound wave is the number of cycles that occurs in 1 second. The unit Hertz is 1 cycle per second. Frequency is an important characteristic of sound in ultrasound imaging as it affects penetration of sound and image quality. In general, higher ultrasound frequencies provide better image quality at the expense of tissue penetration. Power and intensity of the ultrasound beam relate to the strength of a sound wave. Power is the rate of energy transferred through the sound wave and is expressed in Watts. Power can be altered up or down by a control on the ultrasound machine. Intensity is the concentration of energy in a sound wave and thus is dependent on the power and the cross-sectional area of the sound beam. The intensity of a sound beam is thus calculated by dividing the power of a sound beam (Watts) by its cross-sectional area (cm2), expressed in units of W per cm2.
The sound source, which is the ultrasound machine and/or the transducer, determines the frequency, power, and intensity of the sound. The propagation speed of sound in soft tissue is constant at 1,540 m per second. The propagation speed of sound is fastest in bone and is slowest in air. This is why the use of medical ultrasound is limited in anatomic regions involving air, such as the lungs or large bowels.
Sound is classified based upon the ability of the human ear to hear it. Sounds sensed by young healthy adult human ears are in the range of 20 to 20,000 cycles per second or Hertz, abbreviated as Hz, and this range is termed the audible sound (range of 20 to 20,000 Hz). If the frequency of a sound is less than 20 Hz, it cannot be heard by humans and is defined as infrasonic or infrasound. If the frequency of sound is higher than 20,000 Hz or 20 kHz, it cannot be heard by humans and
is called ultrasonic or ultrasound. Typical frequencies used in medical ultrasound are 2 to 10 MHz (mega [million] Hertz). Ultrasound frequencies that are commonly used in obstetrics and gynecology are between 3 and 10 MHz.
is called ultrasonic or ultrasound. Typical frequencies used in medical ultrasound are 2 to 10 MHz (mega [million] Hertz). Ultrasound frequencies that are commonly used in obstetrics and gynecology are between 3 and 10 MHz.
Table 2.1 • Characteristics of a Sound Wave | |||||||
---|---|---|---|---|---|---|---|
|
ULTRASOUND WAVES
Ultrasound waves are generated from tiny piezoelectric crystals packed within ultrasound transducers. When an alternate current is applied to these crystals, they contract and expand at the same frequency at which the current changes polarity and generate an ultrasound beam. The ultrasound beam traverses into the body at the same frequency generated. Conversely, when the ultrasound beam returns to the transducer, these crystals change in shape and this minor change in shape generates a tiny electric current that is amplified by the ultrasound machine to generate an ultrasound image on the monitor. The piezoelectric crystals within the transducer therefore transform electric energy into mechanical energy (ultrasound) and vice versa. A rubber covering on the ultrasound transducer protects the crystal and helps to decrease the resistance to sound transmission (impedance) from the crystals to the body and vice versa. In order to minimize the impact of air, a watery gel is applied on the skin of the patient to facilitate transfer of sound to and from the transducer.
THE ULTRASOUND IMAGE
Modern ultrasound equipment create a graded gray scale ultrasound image by sending multiple sound pulses from the transducer at slightly different directions and analyzing returning echoes received by the crystals. The details of this process are beyond the scope of this chapter, but it is important to note that tissues that are strong reflectors of the ultrasound beam, such as bone or air, will result in a strong electric current generated by the piezoelectric crystals, which will appear as a hyperechoic image (bright) on the monitor (Fig. 2.1). On the other hand, weak reflectors of ultrasound beam, such as fluid or soft tissue, will result in a weak current, which will appear as a hypoechoic or anechoic image (dark) on the monitor (Fig. 2.1). The ultrasound image is thus created from a sophisticated analysis of returning echoes in a gray scale format. Given that the ultrasound beam travels in a longitudinal format, in order to get the best possible image, keep the angle of incidence of the ultrasound beam perpendicular to the object of interest, as the angle of incidence is equal to the angle of reflection.
ULTRASOUND MODES
B-Mode Ultrasound
B-mode ultrasound, which stands for “Brightness mode,” is also known as two-dimensional (2D) imaging and is commonly
used to describe any form of gray scale display of an ultrasound image. The image is created based upon the intensity of the returning ultrasound beam, which is reflected in a variation of shades of gray that form the ultrasound image (Fig. 2.2). It is important to note that B-mode is obtained in real time, an important and fundamental characteristic of ultrasound imaging. B-mode, or gray scale imaging, is the fundamental imaging modality for ultrasound in the first trimester and as discussed later in this chapter, it carries the least amount of energy.
used to describe any form of gray scale display of an ultrasound image. The image is created based upon the intensity of the returning ultrasound beam, which is reflected in a variation of shades of gray that form the ultrasound image (Fig. 2.2). It is important to note that B-mode is obtained in real time, an important and fundamental characteristic of ultrasound imaging. B-mode, or gray scale imaging, is the fundamental imaging modality for ultrasound in the first trimester and as discussed later in this chapter, it carries the least amount of energy.
M-Mode Ultrasound
M-mode ultrasound, which stands for “Motion mode,” is a display that is frequently used in early gestation to assess the motion of the fetal cardiac chambers and valves in order to document cardiac activity. The M-mode originates from a single beam penetrating the body with a high pulse repetition frequency. The display on the monitor shows the time of the M-mode display on the x-axis and the depth on the y-axis (Fig. 2.3).
Spectral (Pulsed) Doppler
Spectral (pulsed) Doppler modes are ultrasound displays that are dependent on the Doppler principle (effect). The Doppler principle describes the apparent variation in frequency of a sound wave as the source of the wave approaches or moves away, relative to an observer. This apparent change in frequency, or what is termed the frequency shift, is proportional to the speed of movement of the sound emitting or reflecting object(s), such as red blood cells within a vessel. This frequency shift is displayed in a graphic form as a time-dependent plot. In this display, the vertical axis represents the frequency shift and the horizontal axis represents the temporal change of this frequency shift as it relays to the events of the cardiac cycle (Fig. 2.4). This frequency shift is highest during systole, when the blood flow is fastest and lowest during end diastole, when the blood flow is slowest in the peripheral circulation (Fig. 2.4). Given that the velocity of flow in a particular vascular bed is inversely proportional to the downstream impedance to flow, the frequency shift therefore derives information on the downstream impedance to flow of the vascular bed under study. The frequency shift is also dependent on the cosine of the angle that the ultrasound beam makes with the targeted blood vessel (see formula in Fig. 2.4). Given that the insonation angle (angle of incidence) is difficult to measure in clinical practice, indices that rely on ratios of frequency shifts were developed to quantitate Doppler waveforms.
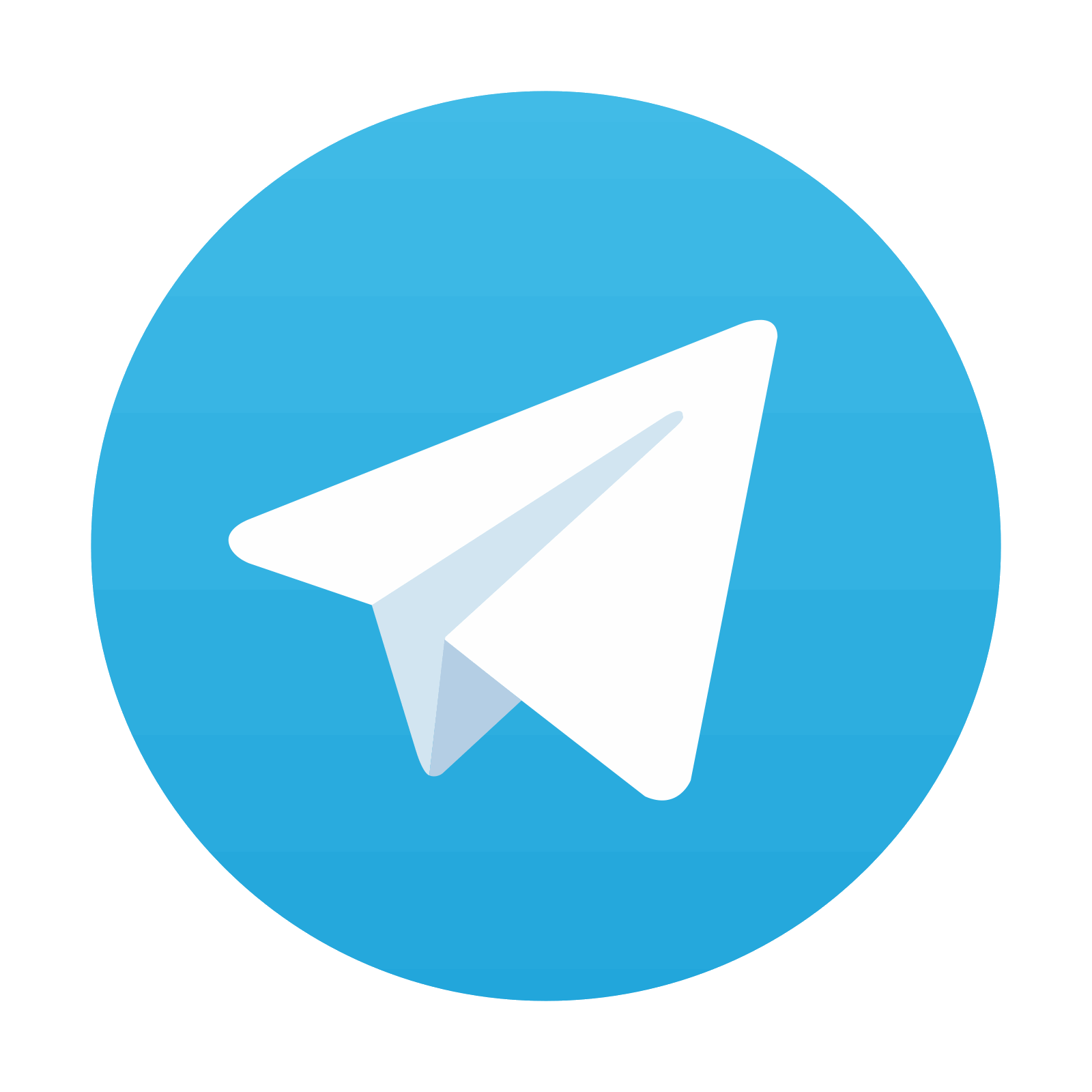
Stay updated, free articles. Join our Telegram channel
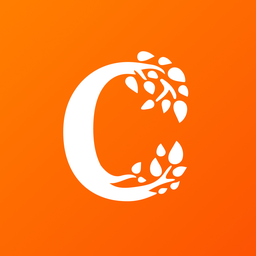
Full access? Get Clinical Tree
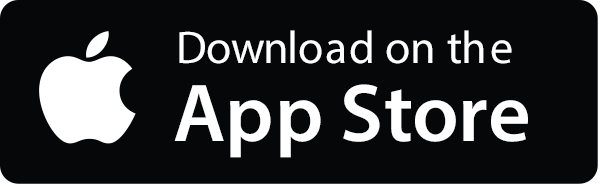
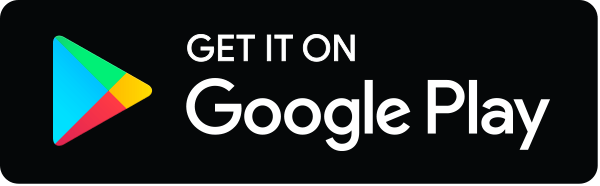