- •
Vascular magnetic resonance imaging is noninvasive, does not involve ionizing radiation, and can provide extensive coverage of large arteries with high accuracy and reproducibility.
- •
As such, it can be used to characterize the composition of atherosclerotic plaques including information on lipid content, fibrous cap integrity, and plaque hemorrhage or thrombosis.
- •
In addition to providing information on vascular structure, MR imaging is also capable of assessing vascular function as part of an integrated examination.
- •
Such measurements of vascular function include arterial pulse wave velocity, aortic compliance and distensibility, and flow-mediated dilatation. Good agreement between ultrasound and MR measurements of these parameters has been proven.
- •
To date vascular magnetic resonance imaging has mainly been used to asses changes in atherosclerotic plaque structure in response to lipid-lowering therapies.
- •
Potential future clinical applications of this technology are continuing to emerge. Furthermore, MR imaging has the potential to capitalize on molecular techniques that may shed new insights into plaque biology.
The walls of large arteries display a spectrum of structural and functional abnormalities in the context of atherogenesis and are, therefore, an attractive target for investigation of this disease process. Paradoxically, atherosclerotic plaque burden has been estimated conventionally by means of invasive X-ray arteriography of the vessel lumen. More recently both carotid ultrasound and intravascular coronary ultrasound have been used to interrogate the arterial wall directly. However, these methods of plaque and wall imaging are also limited. For instance, ultrasound cannot reliably provide detail on plaque composition and morphology unless used invasively (intravascular ultrasound) and is confined to imaging limited segments of arteries, which are accessible to the imaging probe.
By comparison, MR imaging is noninvasive, does not involve ionizing radiation, and can provide extensive coverage of large arteries with high accuracy and reproducibility. As a result, MR imaging can be used to characterize atherosclerotic plaques in the aorta and the carotid arteries, and to measure the response to therapy at multiple vascular sites. Furthermore, in addition to studying vascular structure , MR imaging is capable of assessing vascular function as part of an integrated examination.
Even before the development of macroscopic atherosclerotic lesions, loss of normal arterial elasticity can occur and correlates with established cardiovascular risk factors, and even future risk. Therefore noninvasive measures of arterial “stiffness” ( Table 26-1 ) are of potential use in the assessment of cardiovascular disease. To date these have mostly been assessed using peripheral vascular ultrasound of a superficial artery such as the brachial, but a recent study has demonstrated good agreement between ultrasound and MR measurements of arterial pulse wave velocity ( Case 1 ), compliance and distensibility ( Case 2 ), and flow mediated dilatation ( Case 3 ).
TERM | DEFINITION |
---|---|
Compliance | Absolute change in vessel area for a given change in pulse pressure. |
Distensibility | Relative change in a vessel area for a given change in pulse pressure. |
Pulse Wave Velocity | Velocity of propagation of the physiological pressure pulse wave along an artery. |
Magnetic resonance imaging is well-suited for serial investigations of both vascular structure and function. These assets have been utilized in studies examining the effect of drug intervention ( Case 4 ). Potential new applications of vascular MR continue to emerge, such as its use for the assessment of carotid plaque in acute stroke ( Case 5 ). Finally, the development of imaging probes to allow imaging of atherosclerosis at molecular, cellular, and functional levels has the potential to revolutionize current assessment of vascular disease ( Case 6 ).
Measurement of pulse wave velocity (PWV) between two sites on the arterial tree can give an indication of arterial stiffness ( Figure 26-1 ). As the arterial wall undergoes compositional change, with or without thickening, compliance may be reduced, and the speed of a transmitted arterial pulse wave is increased. This may be accompanied by an increase in systolic blood pressure, pulse pressure widening, and augmentation of late systolic blood pressure. Aortic PWV independently predicts cardiovascular events in the general population, and in patients with diabetes. Furthermore, a reduction in aortic PWV has been linked to a decreased relative risk for all-cause mortality in patients with end-stage renal failure.
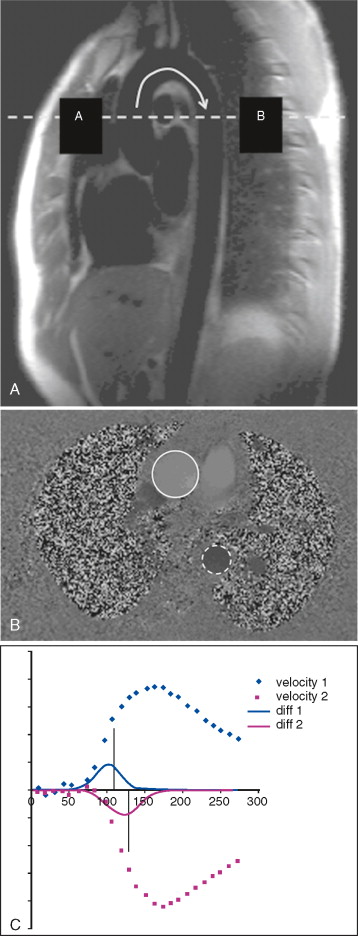
Aortic PWV can be estimated by measuring the velocity of the pulse wave transit from the carotid artery to the femoral artery using ultrasound; however, this technique is limited by several technical factors, such as transducer placement and beam angle. Phase-contrast or velocity-encoded MR allows simultaneous quantification of blood volume through accurately defined and reproducible image planes. To avoid artefact, patient motion must be minimized and the slices must not be positioned near large arterial branches.
Aortic distensibility is defined as the relative change in volume or cross-sectional area for a given change in arterial pressure. The elasticity (and hence the distensibility) of the large arteries is greater proximally because of the higher elastin to collagen ratio in their walls; as a result distensibility is greater in the proximal than the descending aorta. As the elastic fibers of the arteries degenerate with age and in disease, a corresponding increase in arterial stiffness takes place. MR imaging allows careful matching of slice positioning, ensuring that reproducible measurements of aortic distensibility at the same location can be taken ( Figure 26-2 ). Distensibility is reduced in patients with ischemic heart disease and hypertension, and is most accurately calculated when used with central, rather than peripheral, blood pressure measurements. MR imaging can be used to estimate aortic, carotid, and peripheral vascular distensibility noninvasively and with superior reproducibility to ultrasound. Furthermore, in patients with newly diagnosed coronary artery disease initiation of medical therapy, including a statin has been associated with a marked early improvement in aortic distensibility.
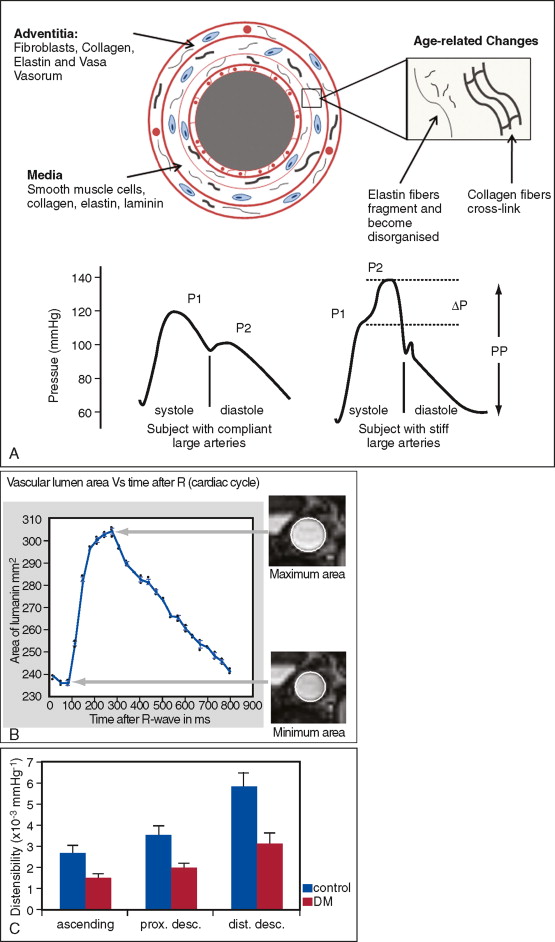
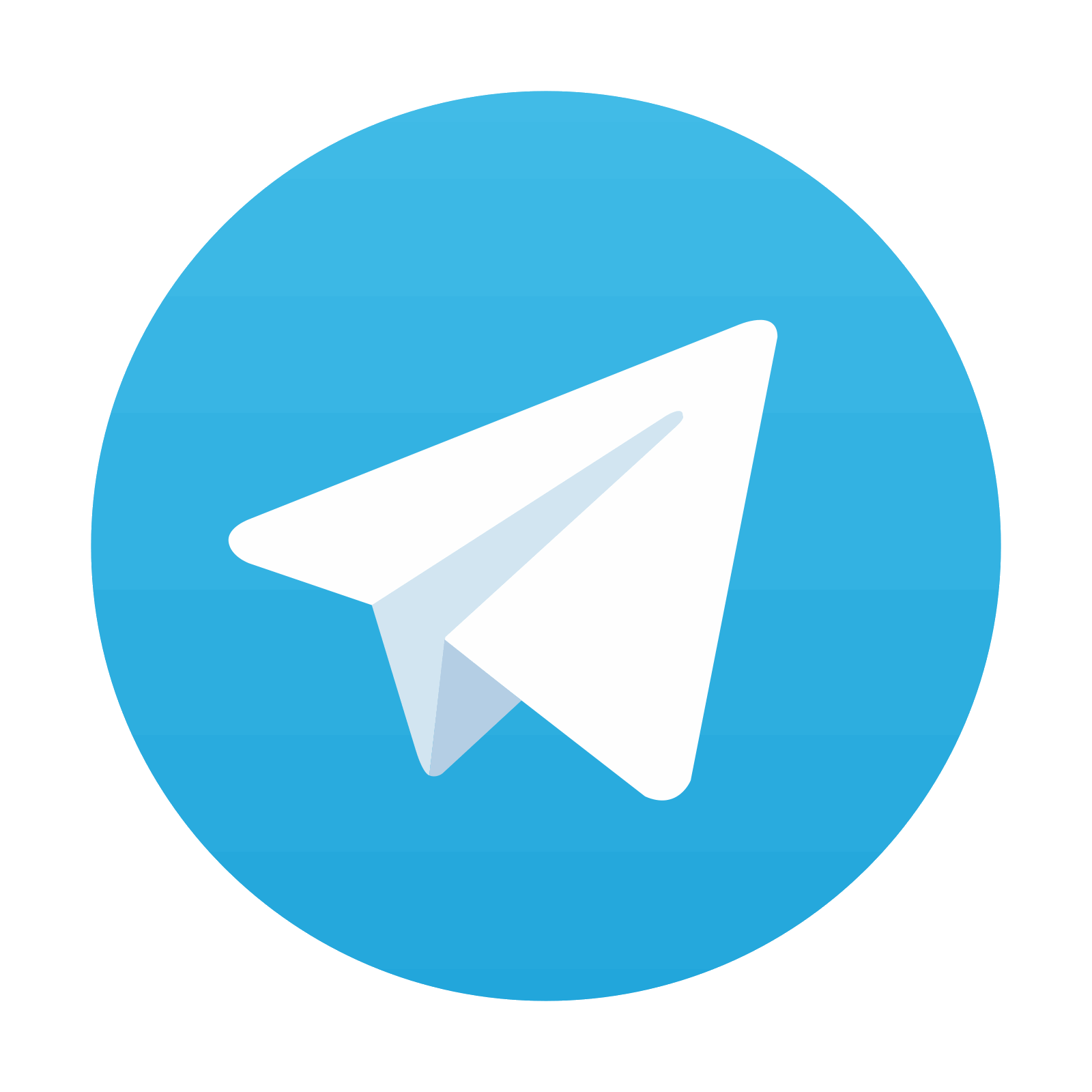
Stay updated, free articles. Join our Telegram channel
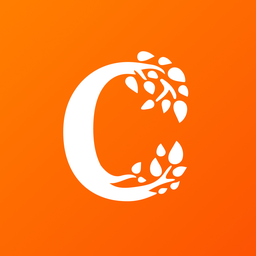
Full access? Get Clinical Tree
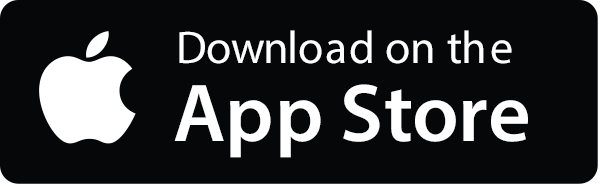
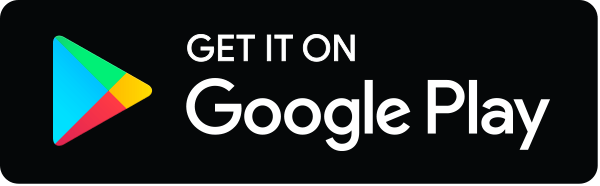
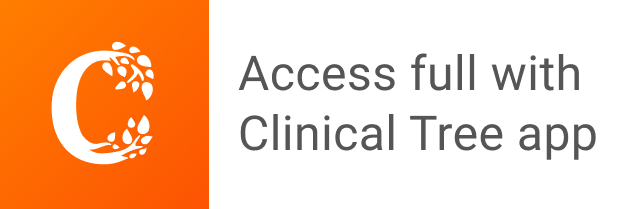