Combined PET/MR imaging scanners capable of acquiring simultaneously the complementary information provided by the 2 imaging modalities are now available for human use. After addressing the hardware challenges for integrating the 2 imaging modalities, most of the efforts in the field have focused on developing MR-based attenuation correction methods for neurologic and whole-body applications, implementing approaches for improving one modality by using the data provided by the other and exploring research and clinical applications that could benefit from the synergistic use of the multimodal data.
Key points
- •
Integrated PET/MR imaging scanners capable of simultaneous data acquisition are commercially available for human use.
- •
In addition to the hardware challenges that needed to be addressed for integrating PET and MR imaging, approaches to overcome specific methodologic hurdles such as the need for deriving the attenuation maps from the MR data had to be implemented.
- •
Methodologic improvements and the synergistic use of the simultaneously acquired datasets are expected to benefit numerous research and clinical applications.
Introduction
Combining PET and MR imaging into a single device capable of simultaneously acquiring both datasets has been of interest for several decades, even before the emergence (and eventual clinical success) of integrated PET and computed tomography (PET/CT) scanners. This integration was motivated by the complementary nature of the information provided by these 2 powerful imaging modalities that are currently widely used for research and clinical applications. On one hand, PET can assay with high molecular sensitivity a wide range of biological processes (from tracking the in vivo distribution of small molecules to cells) using radionuclides that decay by positron emission. However, PET has limited spatial resolution, and most often the images lack the anatomic detail required for clinical interpretation. On the other hand, MR imaging provides high spatial resolution anatomic images with excellent soft tissue contrast even using routine techniques. More advanced MR sequences exploit other sources of endogenous contrast to study physiology (eg, water diffusion), function (eg, blood oxygenation level–dependent contrast), and metabolism (eg, relative concentration of various metabolites), whereas passive contrast agents (eg, gadolinium, iron oxide) that modify the tissue relaxation times can further enhance contrast in the resulting images. The main limitations of MR imaging in this context are that its sensitivity is several orders of magnitude smaller than that of PET and the lack of absolute quantification.
Although software co-registration or a side-by-side analysis can be performed to fuse the PET and MR imaging data acquired separately, this approach eliminates the possibility of temporally correlating the measurements, which could revolutionize several research and clinical applications. Furthermore, in those patients requiring both examinations, the simultaneous acquisition could improve the spatial registration between the 2 images and the workflow and increase the patient’s comfort. For these reasons, this article discusses only those aspects that are relevant to simultaneous acquisition.
Introduction
Combining PET and MR imaging into a single device capable of simultaneously acquiring both datasets has been of interest for several decades, even before the emergence (and eventual clinical success) of integrated PET and computed tomography (PET/CT) scanners. This integration was motivated by the complementary nature of the information provided by these 2 powerful imaging modalities that are currently widely used for research and clinical applications. On one hand, PET can assay with high molecular sensitivity a wide range of biological processes (from tracking the in vivo distribution of small molecules to cells) using radionuclides that decay by positron emission. However, PET has limited spatial resolution, and most often the images lack the anatomic detail required for clinical interpretation. On the other hand, MR imaging provides high spatial resolution anatomic images with excellent soft tissue contrast even using routine techniques. More advanced MR sequences exploit other sources of endogenous contrast to study physiology (eg, water diffusion), function (eg, blood oxygenation level–dependent contrast), and metabolism (eg, relative concentration of various metabolites), whereas passive contrast agents (eg, gadolinium, iron oxide) that modify the tissue relaxation times can further enhance contrast in the resulting images. The main limitations of MR imaging in this context are that its sensitivity is several orders of magnitude smaller than that of PET and the lack of absolute quantification.
Although software co-registration or a side-by-side analysis can be performed to fuse the PET and MR imaging data acquired separately, this approach eliminates the possibility of temporally correlating the measurements, which could revolutionize several research and clinical applications. Furthermore, in those patients requiring both examinations, the simultaneous acquisition could improve the spatial registration between the 2 images and the workflow and increase the patient’s comfort. For these reasons, this article discusses only those aspects that are relevant to simultaneous acquisition.
Technical aspects that must be considered for integrating PET and MR imaging
Considerations on the PET Side
The major technical challenge from the PET perspective is that photomultiplier tubes (PMTs), used until recently in virtually all commercially available PET scanners, are very sensitive to even small magnetic fields and cannot be operated inside modern preclinical or clinical high-field MR devices. To overcome this limitation in the early days of PET/MR imaging, long optical fibers were used to channel the light from the scintillator crystals placed inside the MR system to PMTs located at a safe distance. Several generations of small-animal imaging devices have subsequently been built using PMTs in an effort to increase the PET performance in terms of the spatial resolution uniformity and axial coverage. All these efforts required substantial modification of the PET detectors to maximize compatibility with existing MR imaging devices. Alternatively, minimally modified PET detectors have been integrated in specialized MR imaging scanners (eg, split magnet or field cycled ).
In spite of this important pioneering work, PET/MR imaging would have likely failed to transition from small-animal to human imaging if it were not for the emergence of MR-compatible photon detector technology that allowed the placement of the PET detectors inside the magnet’s bore. Fortunately, solid-state photon detectors have reached a level of maturity that allows them to replace PMTs for this application. Avalanche photodiodes (APDs) were the first such photon detectors that were found to work even inside ultra–high-field magnets and were used to build MR-compatible PET inserts for small animal imaging. More importantly, this technology allowed Siemens to build the first PET/MR imaging prototype scanner for human brain imaging. This device was called BrainPET and was designed to fit into the bore of the standard 3-T whole-body MR imaging scanner (Magnetom Trio, Siemens, Erlangen, Germany). Although only a handful of BrainPET prototypes were ever built and only 3 of them are still in use, these inserts have been used for many studies ranging from those aimed at investigating the mutual interference between the 2 devices and the performance of the APD-based PET detector technology, developing methods to use the information obtained from one device to improve the other modality, and demonstrating the tremendous potential of PET/MR imaging through proof-of-principle studies in small-animal, nonhuman primates and humans.
Additional challenges such as the need to carefully shield the PET detectors to minimize the electromagnetic interference with the MR system or to control for temperature variations in the detectors caused by the eddy currents induced by the switching MR gradients had to be considered when using these solid-state photon detectors.
More recently, Geiger mode APDs (also called solid-state photomultipliers , silicon photomultipliers [SiPMs] or multiphoton pixel counters) have emerged as promising candidates for replacing APDs as the photon detector of choice for simultaneous PET/MR imaging, and several groups have developed small-animal scanners using this technology. There are also now companies (eg, Cubresa, Inc, Bruker Biospin) that offer MR-compatible PET inserts designed to operate inside existing small-animal MR imaging devices.
Considerations on the Magnetic Resonance Side
Simultaneous acquisition requires the placement of PET components (eg, scintillators, photon detectors and associated electronics, cooling components, shielding material) in the bore of the MR scanner. This is a challenge because the main magnetic field homogeneity needs to be maintained for proper MR operation. For this reason, nonmagnetic versions of even standard components (eg, resistors, capacitors, connectors) must be used especially when such components are to be placed close to the subject. Similarly, scintillator materials with a magnetic susceptibility close to that of human tissue (ie, lutetium-based scintillators instead of lutetium gadolinium oxyorthosilicate) are preferred.
Electromagnetic interference in the radiofrequency part of the spectrum has to be minimized to avoid introducing artifacts in the MR images. This minimization is particularly challenging when PET photon detectors and electronics need to be integrated with the MR scanner, as they contain radiofrequency-radiating components. The shielding required to minimize the interference is of special concern because eddy currents can be induced in the shielding enclosures by the changing gradients potentially leading to image artifacts and spatial distortions.
To enable simultaneous acquisition, the MR radiofrequency coils have to be positioned in the PET field of view and thus contribute to photon attenuation. To maximize their PET compatibility, they have to be carefully designed to minimally attenuate the 511 keV photons and any changes must be made in a way that maintains their performance.
The diameter of the bore of most commercially available MR scanners is no larger than 60 cm, which means there is minimal space left to integrate PET components. Fortunately, larger 70-cm-diameter bore scanners were recently introduced by all the 3 major medical equipment manufacturers (ie, Siemens, General Electric, and Philips), and the integrated PET/MR scanners discussed in the next section were built starting from such systems.
Integrated PET/MR imaging hardware for whole-body human imaging
Siemens Biograph mMR
Siemens was the first to introduce a fully integrated whole-body integrated scanner, called Biograph mMR , in 2010 ( Fig. 1 , left). The MR component of the Biograph mMR consists of a 60-cm bore 3-T niobium–titanium superconductor magnet, an actively shielded whole-body gradient coil system (45 mT/m amplitude and 200 T/m/s slew rate), and a radiofrequency body coil. Similar to the BrainPET prototype, the PET component of the mMR also uses APD technology. However, instead of placing the PET detectors inside the MR bore, they are positioned between the body radiofrequency coil and the gradient set. Eight rings of 56 detector blocks make up the whole PET gantry. Each detector block consists of an 8 × 8 array of 4 × 4 × 20 mm 3 lutetium oxyorthosilicate (LSO) crystal readouts by a 3 × 3 array of Hamamatsu APDs. The transaxial/axial fields of view are 59.4/25.8 cm. To address the need for compensating for temperature changes during the MR acquisition, chilled water is used to control the temperature inside the detector modules. In terms of performance, the reported energy resolution is 14.5%, the timing resolution is 2.93 ns, the spatial resolution is 4.3 mm full width at half maximum (FWHM) at 1 cm offset from the center of the field of view, and the scanner sensitivity is 15.0 kcps/MBq. The mMR scanner can acquire list mode data in 3-dimensional mode. The PET images can be reconstructed using either the filtered back projection or the 3-dimensional ordinary Poisson ordered-subset expectation maximization algorithm.
General Electric SIGNA PET/MR Imaging
General Electric introduced the first whole-body SiPM-based integrated PET-MR imaging scanner, called SIGNA PET/MR , in 2013 ( Fig. 1 , right). The MR component of the SIGNA PET/MR scanner consists of a 60-cm bore 3-T superconductive magnet, gradient coils (44 mT/m amplitude and 200 T/m/s slew rate), and transmit/receive body coils similar to the original 3-T MR 750w MR scanner on which it is based. The PET components are placed between the radiofrequency shield of the body coil and the gradient coils. The PET gantry is made up of 28 modules consisting of 20 detector blocks made up of a 4 × 9 array of 4.0 × 5.3 × 25 mm 3 lutetium-based scintillator readout by 1 × 3 arrays of Hamamatsu SiPMs. The transaxial/axial fields of view are 62/25 cm. Chilled water is also used to control the temperature based on the feedback received from 280 thermistors positioned throughout the scanner. In terms of performance, the reported energy resolution is 10.3%, the timing resolution is less than 400 ps, the spatial resolution is 4.4 mm FWHM at 1 cm offset from the center of the field of view, and the scanner sensitivity is 23.3 kcps/MBq.
Magnetic resonance-based PET attenuation correction
The need for deriving the attenuation maps from the MR data was a significant methodologic hurdle that human PET/MR imaging had to face from the very beginning given the different physical principles that underlie the signal generation in the 2 modalities. Specifically, the MR signal depends on proton density and tissue relaxation times and it does not reflect electron density, which is relevant for attenuation correction. This section introduces the standard protocols for generating the attenuation maps available on the Biograph mMR and SIGNA PET/MR imaging scanners, discusses specific aspects that need to be considered in an integrated PET/MR imaging scanner (some of the solutions that have been proposed for addressing these issues are discussed in detail in Yasheng Chen and Hongyu An’s article, “ Attenuation Correction of PET/MR Imaging ,” in this issue), and comment on the potential benefits of using the time-of-flight (TOF) information for improving the accuracy of MR-based attenuation correction methods.
Standard Protocols Approved for Clinical Use
On the Biograph mMR, the attenuation correction is performed using the MR data acquired with a 2-point Dixon volume interpolated breath-hold examination MR sequence. In-phase, out-of-phase, water, and fat images are generated at each bed position and combined to generate the corresponding whole-body images ( Fig. 2 , left). The in-phase images are first thresholded to separate the voxels corresponding to the subject from the background air. An atlas-based approach is next used to segment the lungs. The voxels corresponding to fat and soft tissue voxels are obtained from the fat and water images. Additional postprocessing is performed to remove the skin voxels and noise. Finally, 4 tissue classes (background air, lung, fat, and soft tissue) are segmented, and predefined linear attenuation coefficients (0, 0.022, 0.085, 0.100 cm −1 , respectively) are assigned to generate the attenuation map ( Fig. 2 , right). Bone tissue is misclassified as soft tissue.
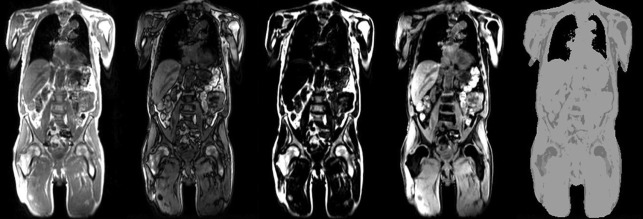
Similarly, on the SIGNA PET/MR imaging scanner, the attenuation information is acquired using a liver acquisition with volume acquisition-flexible (LAVA-Flex) scan and the resulting Dixon images are segmented into air, lung, fat, and water. Bone tissue is again misclassified as soft tissue with the exception of the head, for which an atlas-based registration is used to account for the skull attenuation.
Special Considerations
Although MR-based attenuation correction eliminates the radiation exposure associated with the CT examination in PET/CT scanners (of interest in at-risk patient populations), several practical issues limit the accuracy of the currently available methods.
Bone imaging
Imaging bone tissue is extremely difficult using standard MR sequences, and thus the corresponding voxels cannot be easily identified to be included in the attenuation map. However, bone tissue has the highest linear attenuation coefficients and cannot be ignored without biasing the PET measurements.
Truncation artifacts
Because of the long duration of a comprehensive whole-body PET/MR imaging examination, the patients are scanned with their arms in the field of view. In MR imaging, geometric distortions are present in the case of large fields of view (ie, >∼45 cm diameter), which results in complete or partial truncation of the arms or other body regions and, consequently, of the attenuation maps derived from these MR images. As a result, bias in PET quantification could be introduced, potentially compromising the clinical interpretability of the PET images. On the Biograph mMR scanner, a maximum likelihood activity and attenuation estimation algorithm was implemented to recover the missing data. At each iteration, first the activity is updated, maintaining the linear attenuation coefficients constant, and second the attenuation map is updated while keeping the emission image constant. When successful, this method was shown to reduce the error in the standardized uptake values estimation from 15% to 50% to less than 5%. A similar algorithm that uses the nonattenuation-corrected TOF-reconstructed PET image to estimate the patient outline is available on the SIGNA PET/MR imaging scanner.
Metallic implants
Foreign objects present in the body (eg, prosthetic devices, dental implants, subcutaneous chemotherapy ports, and surgical clips) can introduce susceptibility artifacts in the MR images, leading to signal voids in or distortions of the MR images that propagate in the attenuation maps derived from these data. Novel MR sequences have been proposed to minimize the susceptibility artifacts in the MR images in the presence of metallic implants and they could minimize this source of bias.
Lung imaging
Imaging the lung parenchyma using conventional MR sequences is extremely challenging because of its very short T 2 * (ie, 0.74 ms at 3-T ), low proton density, and subject motion. However, there is large variability in lung attenuation, not only when comparing diseased versus normal tissue but also in the different phases of the respiratory cycle.
Magnetic resonance hardware
Although the radiofrequency coils have to be redesigned and built using minimally attenuating materials, not accounting for their remaining attenuation in the correction procedure could still bias the PET measurements. The attenuation templates for several of the standard coils (eg, head and neck coil, spine) are provided by the manufacturers whereas the flexible body coils are ignored in the attenuation correction procedure.
The Potential Added Value of the Time-of-Flight Information
The major difference between the 2 integrated PET/MR imaging scanners that are currently commercially available is that the SIGNA PET/MR scanner has TOF capability. The main advantage of incorporating the TOF information is that the signal-to-noise ratio improves compared with the non-TOF images. The various ways in which this can benefit clinical PET studies (eg, improved image quality and lesion detection, shorten the scan time, or reduce the administered dose) have been discussed extensively in the context of PET/CT.
Although both vendors seem to agree on the value of TOF in the case of integrated PET/CT scanners, there is still a debate on this topic when it comes to PET/MR imaging. In the context of MR-based attenuation correction, the TOF information might prove useful for mitigating some of the remaining challenges and artifacts described above.
The standard MR-based attenuation correction methods and most of the more advanced ones presented in Yasheng Chen and Hongyu An’s article, “ Attenuation Correction of PET/MR Imaging ,” in this issue, share the same limitation—using only the MR data and templates of linear attenuation coefficients it is impossible to account for large intersubject variability. One approach to overcome this limitation is to incorporate the information inherently present in the PET emission data. This is because the anatomic information provided by the MR or the TOF minimizes the attenuation-emission estimation cross-talk that has limited the usefulness of the early approaches (ie, any errors in the estimation of emission image lead to compensatory errors in the estimation of the attenuation image). For example, a method similar to the maximum likelihood activity and attenuation discussed above for addressing the arms truncation problem and the TOF information were used to improve the estimation of the linear attenuation coefficients assigned to the different regions segmented from the MR data.
Several recent studies found the TOF can reduce the effect of inconsistencies between the emission and attenuation data, such as those encountered in MR-based attenuation correction caused by bone or lung tissue misclassification, incomplete attenuation maps caused by truncation, or implant-related signal voids.
For example, Mehranian and Zaidi used CT data to generate 4 class-segmented attenuation maps that were used to reconstruct the PET data with and without using the TOF information. They noted significant changes between the 2 methods in vertebra, pelvis, and cerebrum but not in the lungs, aorta, and liver ( Fig. 3 ). The TOF reconstruction was also able to reduce the artifacts related to metallic implants and those introduced by respiratory motion. Although TOF could theoretically reduce the effect of some of these artifacts, the actual impact is still limited given the TOF performance of current generation PET/MR imaging scanners.
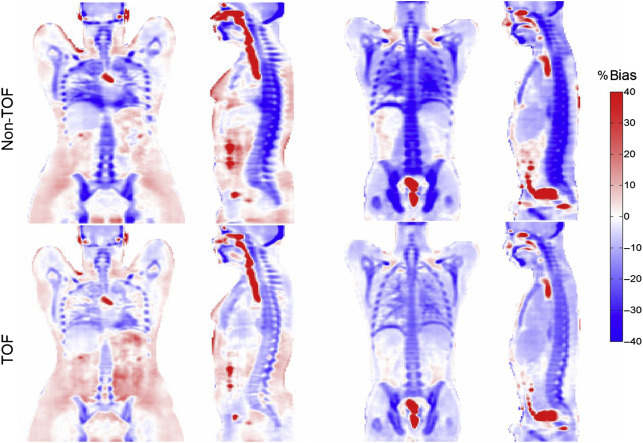
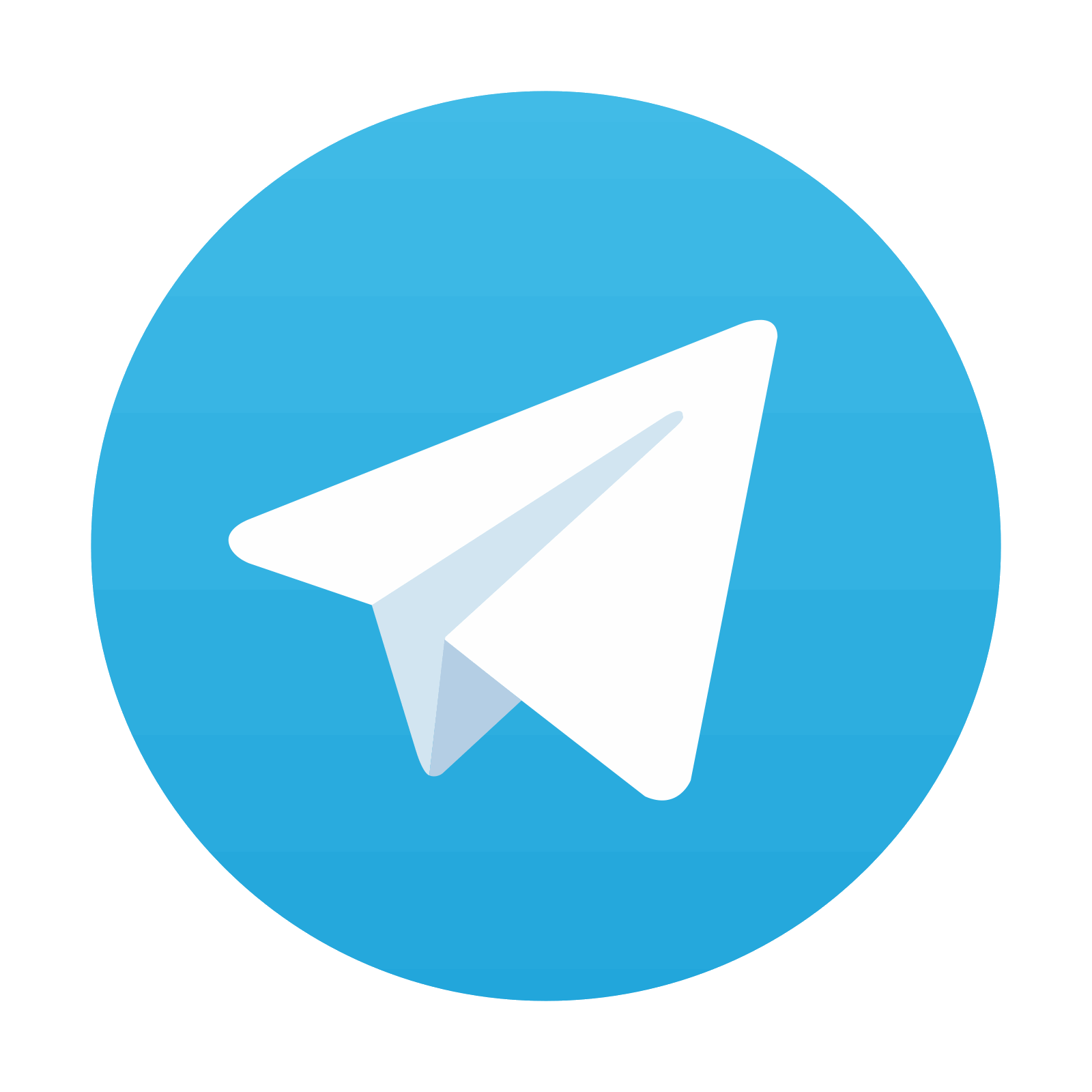
Stay updated, free articles. Join our Telegram channel
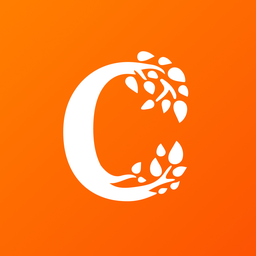
Full access? Get Clinical Tree
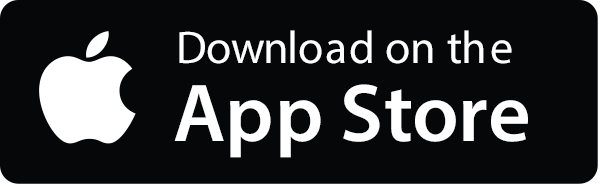
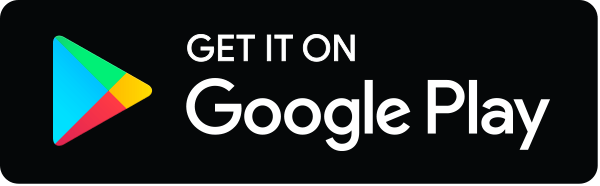
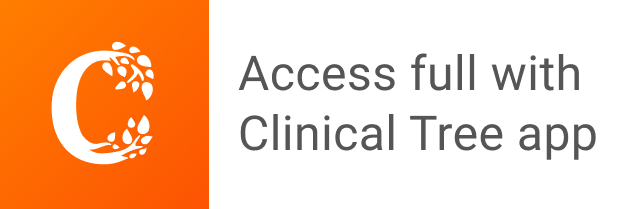