Production of Radionuclides for PET
Ronald D. Finn
David J. Schlyer
Coupled with the advancement in noninvasive cross-sectional imaging techniques to identify structural alterations in diseased tissues, there have been significant advances in the development of in vivo methods to quantify functional metabolism in both normal and diseased tissues. Positron emission tomography (PET) is an imaging modality that yields physiologic information necessary for clinical diagnoses based on altered tissue metabolism.
One of the most widely recognized advantages of PET is the use of the positron-emitting biologic radiotracers (carbon-11 [11C], oxygen-15 [15O], nitrogen-13 [13N], and fluorine-18 [18F]) that mimic natural substrates. These radionuclides have well-documented nuclear reaction cross sections appropriate for “baby” cyclotron energies, and the corresponding “hot atom” target chemistries are reasonably well understood. A disadvantage these biologic radionuclides possess is their relatively short half-lives, which means they cannot be transported to sites at great distances from the production facility.
Currently, there are four PET drugs officially recognized by the U.S. Food and Drug Administration (FDA) and approved for intravenous injection. They are sodium fluoride (18F) (previously FDA approved and currently United States Pharmacopeia [USP] listed), rubidium-82-chloride (82Rb), 13N-ammonia and fluorodeoxyglucose (18F-FDG). In 1972, 18F (New Drug Application [NDA] 17-042) was approved as an NDA for bone imaging to define areas of altered osteogenic activity, but the manufacturer ceased marketing this product in 1975. Rubidium-82-chloride (NDA 19-414) was approved in 1989 and is indicated for assessment of regional myocardial perfusion in the diagnosis and localization of myocardial infarction. Most recently, 18F-FDG (NDA 20-306) was recognized in 1994 for identification of regions of abnormal glucose metabolism initially associated with foci of epileptic seizures, but it is now mostly used and approved for its application to various primary and metastatic malignant diseases (1). Nitrogen-13-ammonia is approved for assessment of myocardial blood flow.
Over the past few decades, PET studies with radiolabeled drugs have provided new information on drug uptake, biodistribution, and various kinetic relationships. A critique on the design and development of PET radiopharmaceuticals has been published (2), as well as several articles involving the future of PET in drug research and development and the production targetry available from various manufacturers of cyclotrons (3). Growth in clinical PET applications has led to increased interest in and demand for new PET radiopharmaceuticals.
Production
Definition of Nuclear Reaction Cross Section
A nuclear reaction is one in which a nuclear particle is absorbed into a target nucleus, resulting in a very short-lived compound nucleus. This excited nucleus will decompose along several pathways and produce various products. A wide variety of nuclear reactions are used in an accelerator to produce artificial radioactivity. The bombarding particles are usually protons, deuterons, or helium particles. The energies used range from a few million electron volts to hundreds of million electron volts. One of the most useful models for nuclear reactions is the compound nucleus model originally introduced by Bohr in 1936. In this model, the incident particle is absorbed into the nucleus of the target material and the energy is distributed throughout the compound nucleus. In essence, the nucleus comes to some form of equilibrium before decomposing and then emitting particles. These two steps are considered to be independent of each other. Regardless of how the compound nucleus got to the high-energy state, the decay of the radionuclide will be independent of the way in which it was formed. The total amount of excitation energy contained in the nucleus will be given by the following equation:
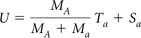
where U equals excitation energy, MA equals the mass of the target nucleus, Ma equals the mass of the incident particle, Ta equals kinetic energy of the incident particle, and Sa equals the binding
energy of the incident particle in the compound nucleus. The nucleus can decompose along several channels, as shown in Fig. 1.1.
energy of the incident particle in the compound nucleus. The nucleus can decompose along several channels, as shown in Fig. 1.1.
When the compound nucleus decomposes, the kinetic energy of all the products may be either greater or less than the total kinetic energy of all the reactants. If the energy of the products is greater, the reaction is said to be exoergic. If the kinetic energy of the products is less than that of the reactants, the reaction is said to be endoergic. The magnitude of this difference is called the Q value. If the reaction is exoergic, Q values are positive. An energy-level diagram of a typical reaction is shown in Fig. 1.2.
The nuclear reaction cross section represents the total probability that a compound nucleus will be formed and that it will decompose in a particular channel. There is a minimum energy below which a nuclear reaction will not occur except by tunneling effects. The incident particle energy must be sufficient to overcome the coulomb barrier and to overcome a negative Q value of the reaction. Particles with energies below this barrier have a very low probability of reacting. The energy required to induce a nuclear reaction increases as the Z of the target material increases. For many low-Z materials it is possible to use a low-energy accelerator, but for high-Z materials it is necessary to increase the particle energy (4).
![]() Figure 1.2. Energy level diagram for a nuclear reaction. The Q value is the difference in the energy levels of the reactants and the products. |
The following relationship (4) gives the number of reactions occurring in 1 second:

where dn is the number of reactions occurring in 1 second, I0 is the number of particles incident on the target in 1 second, NA is the number of target nuclei per gram, ds is the thickness of the material in grams per centimeter squared, and ρab is the parameter called the cross section expressed in units of centimeters squared. In practical applications, the thickness ds of the material can be represented by a slab of thickness Δs thin enough that the cross section can be considered as constant. NA ds are then the number of target atoms in a 1-cm2 area of thickness Δs. If the target material is a compound, rather than a pure element, then the number of nuclei per unit area is given by the following expression:

where NA is the number of target nuclei per gram, FA is the fractional isotopic abundance, C is the concentration in weight, ![]() |
This leads to one of the basic facts of life in radioisotope production. It is not always possible to eliminate the radionuclidic impurities even with the highest isotopic enrichment and the widest energy selection. An example of this is given in Fig. 1.3 for the production of iodine-123 (123I) with a minimum of iodine-124 (124I) impurity (5,6,7,8).
As can be seen from Fig. 1.3, it is not possible to eliminate the 124I impurity completely during the 123I production since the 124I is being concurrently formed at the same energy. To minimize the 124I impurity, irradiation of the target at an energy where the production of 124I is near a minimum becomes an option. In this case a proton energy higher than 20 MeV will give a minimum of 124I impurity.
Enriched Targets
Although they generally play a supplementary role to the applications in the production of radionuclides, stable isotopically labeled compounds find widespread use in pharmacologic and toxicologic investigations. Their use as internal standards in such sensitive and specific analytical techniques as gas chromatography-mass spectroscopy and high-pressure liquid chromatography coupled with mass spectroscopy is of great benefit in the assay of body fluids. Paramagnetic stable nuclides such as carbon-13 (13C) offer opportunities for nuclear magnetic resonance (NMR) analyses of biological samples and possibly whole-body NMR in metabolic studies (9,10,11).
Stable isotopes have for many years been the foundation for the production of radionuclides when pure radionuclides are necessary. Since the invention of the “cyclotron” by Professor E.O. Lawrence in 1929 and proof of acceleration by M.S. Livingston in 1931, the accelerators have provided unique radionuclides for numerous applications.
In the past decade there has been a significant increase in the acquisition and use of “small” cyclotrons devoted principally to operation by chemists for the production of the biomedically useful radiolabeled compounds or radiopharmaceuticals. The primary impetus has been the acceptance of the potential of PET as a dynamic molecular imaging technique applicable to clinical diagnoses while providing the opportunity to evaluate novel radiotracers and radioligands for monitoring in vivo biochemical or physiologic processes with exquisite sensitivity.
Concurrent with the growth of PET/cyclotron facilities has been an emphasis on the production of larger amounts of the short-lived radionuclides in a chemical form suitable for efficient synthetic application. The radionuclidic purity of the final nuclide is an important concern. Targetry and target chemistry continue to be factors for the synthetic chemist’s consideration and appreciation of material science and radiation chemistry effects.
With energy constraints imposed by the various accelerators chosen for installation in imaging facilities, the availability and the application of stable enriched target materials for the production of the biologically equivalent radionuclides is of paramount concern. The calutrons at Oak Ridge National Laboratory are no longer in service to prepare and provide the numerous stable enriched nuclides needed for the variety of radionuclides being evaluated for clinical applications. These concerns still plague many investigators who have experienced the lack of or shortages in availability of such important target materials. A current example involves H218O for 18F production. The H218O target is the choice of most centers for the production of 18F-labeled fluoride anion used in most 18F-labeled radiopharmaceutical production (12,13). Fortunately, other sources have come forward to provide the H218O target as use of 18F has increased.
Targets and Irradiation
Traditional PET Radioisotopes
There are four positron-emitting radioisotopes that are considered the biologic tracers and their clinical and investigational uses are extensive. The radionuclides are 18F, 11C, 13N, and 15O. The reason these are so commonly used is that they can be easily substituted directly onto biomolecules. Carbon-11, 13N, and 15O are the “elements of life.” Substitution of 11C for carbon-12 (12C) does not significantly alter the reaction time or mechanisms of a molecule. A similar situation exists for 13N and 15O. Fluorine-18 can often be substituted for a hydroxy group in a molecule or placed in a position where its presence does not significantly alter the biological behavior of the molecule. When the nucleus decays, the positron emitted will slow to thermal energies and annihilate upon interaction with an electron to produce two 511 keV gamma rays emitted at nearly 180 degrees to each other. The decay characteristics of the positron-emitting radionuclides allow the physiologic processes occurring in vivo to be quantitated by detectors outside the body. Physiologic modeling can be carried out using this information, and quantitative assessments of the biologic function can be made.
Target Irradiation
The positron-emitting radionuclides are produced during the target irradiation and converted to a synthetic precursor, either in the target or immediately after exiting the target. The precursor is next converted into the molecule of interest. This chapter covers only the targetry and the formation in the target of the chemical compound. The formation of precursors outside the target and the conversion of these precursors to the desired radiotracer are covered in Chapter 2. Most of the targets for the production of the biologic radionuclides have been either gases or liquids, although several solid targets have also been developed.
The number and type of products that are obtained in a target are a function of the irradiation conditions, the mixture of gases or liquids in the target, and the presence of any impurities in the target or gas mixture. Changing the chemical composition or physical state of the target during irradiation can alter the chemical form of the final product (14). These are all results of the “hot atom” chemistry and radiolysis occurring in the target during the irradiation. Hot atom is the term used to identify atoms with excessive thermal or kinetic energy or electronic excitation. When an atom undergoes
a nuclear transformation, it usually has a great deal of excess energy imparted from the incident bombarding particle and perhaps from the nuclear reaction. This energy can be manifested in any or all of the normal modes of excitation, including rotational, translational, or electronic. In nearly all cases, the amount of energy present is sufficient to break all the existing chemical bonds to the atom and to send the newly transformed atom off with high kinetic energy. This energy is called the recoil energy, and as the atom slows down, it imparts this energy to the surrounding environment. After the atom has transferred most of its excess energy to the surroundings and slowed to near thermal energies, it usually reacts chemically with the surroundings to form a compound. This compound may be stabilized or may undergo further reactions to form other chemical products.
a nuclear transformation, it usually has a great deal of excess energy imparted from the incident bombarding particle and perhaps from the nuclear reaction. This energy can be manifested in any or all of the normal modes of excitation, including rotational, translational, or electronic. In nearly all cases, the amount of energy present is sufficient to break all the existing chemical bonds to the atom and to send the newly transformed atom off with high kinetic energy. This energy is called the recoil energy, and as the atom slows down, it imparts this energy to the surrounding environment. After the atom has transferred most of its excess energy to the surroundings and slowed to near thermal energies, it usually reacts chemically with the surroundings to form a compound. This compound may be stabilized or may undergo further reactions to form other chemical products.
Several distinguishing characteristics set these types of reactions apart from other chemical reactions. These include reactions that are (a) insensitive to the temperature of the surroundings, (b) independent of the phase of the reaction, (c) dependent on the radical scavengers present in the medium, and (d) dependent on moderators in the medium, such as inert gases (15). There have been several excellent reviews concerning the topic of hot atom chemistry (16,17,18).
Specific Activity
Another topic of importance in the preparation of radioisotopes is that of specific activity. It is important in several applications, and particularly important in PET, where the radionuclide is incorporated into a radiotracer that is used to probe some physiologic process in which very small amounts of the biomolecule are being used. PET is basically a tracer method, and the goal of the PET experiment is to probe the physiologic process without perturbing that process. If the amount of radiotracer is very small in comparison to the amount of the native compound or its competitor, then the process will be perturbed very little. When carrying out studies such as probing the number of receptors or probing the concentration of an enzyme, either of which may be present in very small quantities, these considerations become even more important (19).
Table 1.1 Decay Characteristics for Specific PET Radionuclides | |||||||||||||||||||||||||||||||||||||||||||||||||||||||||||||||||||||||||||||
---|---|---|---|---|---|---|---|---|---|---|---|---|---|---|---|---|---|---|---|---|---|---|---|---|---|---|---|---|---|---|---|---|---|---|---|---|---|---|---|---|---|---|---|---|---|---|---|---|---|---|---|---|---|---|---|---|---|---|---|---|---|---|---|---|---|---|---|---|---|---|---|---|---|---|---|---|---|
|
The usual way to express the concept of specific activity is in terms of the amount of radioactivity per mole of compound. There is, of course, an ultimate limit, which occurs only when the radioactive atoms or radiolabeled molecules exist. Table 1.1 lists the characteristics of the PET radionuclides presented in this chapter (20).
As an example, typical specific activities for 11C-labeled molecules being reported are on the order of 10 Ci/μmol (370 GBq/μmol). Therefore, it can be appreciated that only 1 in 1,000 of the radiotracer molecules is actually labeled with 11C. The rest contain stable 12C. The specific activity is important in probing areas such as receptor binding, enzyme reaction, gene expression, and, in some settings, antigen binding with radiolabeled monoclonal antibodies.
In the area of monoclonal antibody labeling, there is the problem of the incorporation of the label into the molecule. If there is excessive carrier, then a smaller amount of the radiolabel will be incorporated into the molecule. This means that a diagnostic radioisotope may be more difficult to visualize or the dose of a therapeutic radioisotope to the target organ may be less than could be achieved. In addition, too many substitutions on the antibody molecule may reduce its immunological functionality. The specific activity of other PET tracers has been explored extensively. Some recent issues are the specific activity of radiotracers produced from the stable species (21), bromine-76 (76Br) (22), and 13N-labeled ammonia (23).
The radionuclide on which more effort has been expended in attempts to control specific activity is 11C, and we use it here as an example of the things that may be done to maximize the specific activity. Carbon-11 is a challenging radioisotope for achieving high specific activity because carbon is so ubiquitous in the environment. There can never be a truly carrier-free radiotracer labeled with 11C, but only one in which no carrier carbon has been added and steps have been taken to minimize the amount of carbon that can enter the synthesis from outside sources. There can never be less carbon incorporated into the molecule than there is carbon present in the target during the irradiation to produce 11C. It is critical to use the highest possible purity of nitrogen gas in the target and to ensure that the target is absolutely as gas tight as possible.
The walls of the target can also influence the specific activity because many alloys used to fabricate targets contain traces of carbon from the manufacturing process. During irradiation, these traces of carbon can make their way out of the target walls and into the gas phase where they will be incorporated into the final product. A correlation between the target surface area and the mass of carbon introduced into the synthesis has been observed and documented (24,25). Solvents used to clean the metal surfaces or oils left
over from the fabrication process can also serve as sources for carbon in the targets. The input and output lines can also have the same or similar contaminants, and such equipment as valves, connectors, insulators, regulators, and flow controllers all can contribute to the carrier carbon, and care must be taken to minimize the carbon added from these sources.
over from the fabrication process can also serve as sources for carbon in the targets. The input and output lines can also have the same or similar contaminants, and such equipment as valves, connectors, insulators, regulators, and flow controllers all can contribute to the carrier carbon, and care must be taken to minimize the carbon added from these sources.
All the chemical reagents used in the synthesis may also add carrier carbon and must be scrutinized to minimize this contribution.
Fluorine-18
Fluorine-18 has a 109.8-minute half-life and decays 97% by positron emission. The other 3% is by electron capture. It forms very strong covalent bonds with carbon compounds and can be incorporated into a wide variety of organic molecules. It can be substituted for a hydroxy group, as in the case of deoxyglucose, or can be substituted for a hydrogen atom. The van der Waals radius of the fluorine atom is similar to that of the hydrogen atom; therefore, substitution of fluorine for hydrogen causes very little steric alteration of the molecule. The concern with the fluorine for hydrogen substitution is that the electronegative nature of fluorine can alter the electron distribution in a way that will alter the binding properties of a molecule. In some ways, however, fluorine is the most attractive of the four positron emitters commonly used in organic synthesis. The low energy of the positron gives the highest potential resolution for PET imaging. The range of the positrons with average energy in water is much less than 2 mm. The nearly 2-hour half-life allows for a more complex synthesis to be carried out within the decay time of the radioisotope. The electronic perturbation has also sometimes resulted in a molecule that has more physiologically desirable properties than the original compound.
The most widely used radiotracer in PET by far is 2-[18F]fluoro-2-deoxyglucose (18FDG). It has proven to be of great utility in the measurement of the “rate” of glycolytic metabolism in a wide variety of organs and disease states in humans.
Production Reactions for Fluorine-18
There are a number of nuclear reactions that can be used to produce 18F. The major routes are the 18O(p,n)18F reaction (26), usually carried out on oxygen-18 (18O)-enriched water or oxygen gas, and the 20Ne(d,α)18F reaction (27). A number of other reactions are being used, but these two are the principal routes to 18F.
The cross sections for these reactions have been explored extensively and the values are well characterized. The most common reaction in routine applications is the proton reaction on enriched 18O. The yield is significantly higher than the other reactions, and the availability of low-energy proton accelerators has made this the reaction of choice, even in the face of the cost of the enriched 18O target material. The other common reaction, particularly for the production of electrophilic fluorine, is the 20Ne(d,α)18F reaction on natural neon. The yield from this reaction is substantially less, but the ability to add other chemical constituents and the natural abundance of the target material are advantages (28,29,30).
Targetry for Fluorine-18
The number and types of targets that have been designed and fabricated for the production of 18F are very large. There have been several reviews of the types of targets (28,29,30,31,32). For descriptive purposes, the targets can be divided into three basic categories: (a) the gas target primarily used for the production of electrophilic fluorine, (b) the liquid target, usually used for production of 18F-fluoride, and (c) the solid targets, which are not commonly used for the production of 18F.
For gaseous targets, there are two basic considerations. The first is the neon gas target. This target was used for many years for the production of F218F from the 20Ne(d,α)18F reaction (27,28). In this target, a small amount of fluorine gas, typically 0.1% to 0.2%, is added to the neon gas before irradiation. The design of the target has undergone significant changes from the first targets to the current design. Early targets were made of nickel or nickel alloys. The reason for this choice was because it was known that nickel parts would withstand a fluorine atmosphere, and most fluorine handling systems were made from nickel or alloys such as Inconel or Monel (Special Metals Corp, Huntington, West Virginia), which have a high nickel content. It was later shown that any surface that could be passivated by fluorine could be used in the fluorine target (33). This discovery introduced the possibility of using aluminum target bodies for the production of elemental fluorine. The activation properties of aluminum are vastly superior to those of nickel or steel in terms of avoidance of the long-lived activities, which are produced within the target body during the bombardment. Target bodies constructed of aluminum significantly reduce the radiation dose received by the technical staff during the cleaning and maintenance of the target. A more extensive investigation of the properties of the surface has been made (30,34). It was shown that aluminum, copper, and nickel form fluoride layers and therefore passivate. The metal surfaces may also contain oxide layers. Only gold does not form a fluoride layer. Exposure to air after passivation does not alter the surface layer (33,34).
The direct addition of fluorine to the neon before irradiation was one method for the recovery of the fluorine in elemental form. The other method was developed by Nickles et al. (35) and is called the two shoot method. In this method, the fluorine is allowed to stick to the walls of the target during the irradiations and is then removed by creating a plasma containing elemental fluorine, which reacts with the 18F on the walls and brings it into the gas phase. The usual gas for this target is the 18O-enriched O2 gas. Other methods for converting the 18F in other chemical forms such as hydrogen fluoride (HF) to F2 outside the target have also been attempted, but with limited success (36,37). In this latter case, the neon or 18O-enriched oxygen gas is irradiated and the fluorine allowed to stick to the walls. In some cases, hydrogen is added to the target gas during irradiation. After irradiation, the target gas is removed, and then the target is heated and flushed with hydrogen to bring the fluorine out in the form of HF (3). The production of other fluorinating intermediates has also been described by using in-target chemistry, but these are not currently in widespread use (38).
A high-energy reaction of protons on neon can also be used in the same way as the deuterons on neon (39,40). The fluorine can be brought out of the target in the form of fluoride ion if the target is washed after irradiation with an aqueous solution (29,31), or the glass liner of the target can be used directly as the reaction vessel (35). In all cases, the fluorine is recovered from the surface in relatively high yields (more than 70%). Whether the protons on 18O or neon, or the deuterons on neon reaction, is used, the result and methodology are essentially the same.
By far the most commonly used target compound for the production of 18F in the form of fluoride ion is the H218O target. The basic design is relatively straightforward and similar to most of the targets being used routinely. There are wide variations, however, in
the details of the design and the construction materials (41,42,43,44,45,46,47,48,49,50,51,52,53). The primary constraint is to use as little of the H218O as possible while leaving enough volume to take maximum advantage of the cross section and to absorb or transfer the heat created by the passage of the beam. A typical target is shown in Fig. 1.4.
the details of the design and the construction materials (41,42,43,44,45,46,47,48,49,50,51,52,53). The primary constraint is to use as little of the H218O as possible while leaving enough volume to take maximum advantage of the cross section and to absorb or transfer the heat created by the passage of the beam. A typical target is shown in Fig. 1.4.
There are several considerations in the operation of the target. The first is the fact that the water would boil due to high temperatures generated by irradiation unless the pressure in the target is increased to diminish or inhibit the boiling (54,55,56). To reduce this problem, the target may be run under elevated pressure of helium, nitrogen, or some other inert gas, or the target may be valved off and allowed to find its own pressure level. In this case, pressure can exceed 40 atm, particularly if the water has not been completely degassed before use. Because a relatively thin foil contains the pressure, there is a limit to the beam current that can be applied in this situation.
The decision to operate at low or high pressure will also have an impact on the target fabrication and the materials chosen for the target. The radiolysis products of the water will have different effects depending on the conditions inside the target. The materials used to construct the target can also have an effect on the chemical reactivity of the fluoride obtained from the target (57,58,59). If the target is operated at low pressure, there will be some loss of the water out of the beam strike area due to bubble formation (45,54).
There have been some unique designs for the water target using spherical targets (60) or flowing targets (46) or frozen 18O-enriched carbon dioxide targets (61). The helium-3 or α-reaction on natural water has also been used to produce 18F for synthesis (8,62,63). These targets work exactly the same way as the proton on water targets, except that the level of heat deposition is higher with the heavier particles. These targets are not commonly used because the yields are substantially lower.
Radioisotope Separation for Fluorine-18
There are two separate scenarios for recovery of 18F from the target, which depend on the mode of production. In the case of the gas target, the fluorine (with the carrier F2) is removed from the target as a gas mixture and can be used in the synthesis from there. In the case of the water target, the activity is removed in the aqueous phase. There are two general methods after that. The first is to use the H218O-containing 18F-fluoride ion directly in the synthesis. This method is used by several investigators who have small-volume water targets, and the cost of losing the H218O is minor compared with the cost of the cyclotron irradiation. The other method is to separate the fluoride from the H218O, either by distillation or by using a resin column (64,65,66). When the resin is used, it also separates the metal ion impurities from the enriched fluoride solution. This resin purification generally increases the reactivity of the fluoride.
Carbon-11
Carbon-11 has a 20.4-minute half-life and decays 99.8% by positron emission and only 0.2% by electron capture. It decays to stable boron-11. Carbon-11 offers the greatest potential for the synthesis of radiotracers that track specific processes in the body. The short half-life of 11C limits processes that can be adequately studied. The chemical form of 11C can vary depending on the environment during irradiation. The usual chemical forms of 11C obtained directly from the target are carbon dioxide and methane.
Production Reactions for Carbon-11
There are several reactions used to produce 11C. By far the most common reaction is the 14N(p,α)11C reaction on nitrogen gas (67,68). This reaction produces a high yield of 11C, and with the addition of trace amounts of oxygen, 11C is almost exclusively in the chemical form of carbon dioxide.
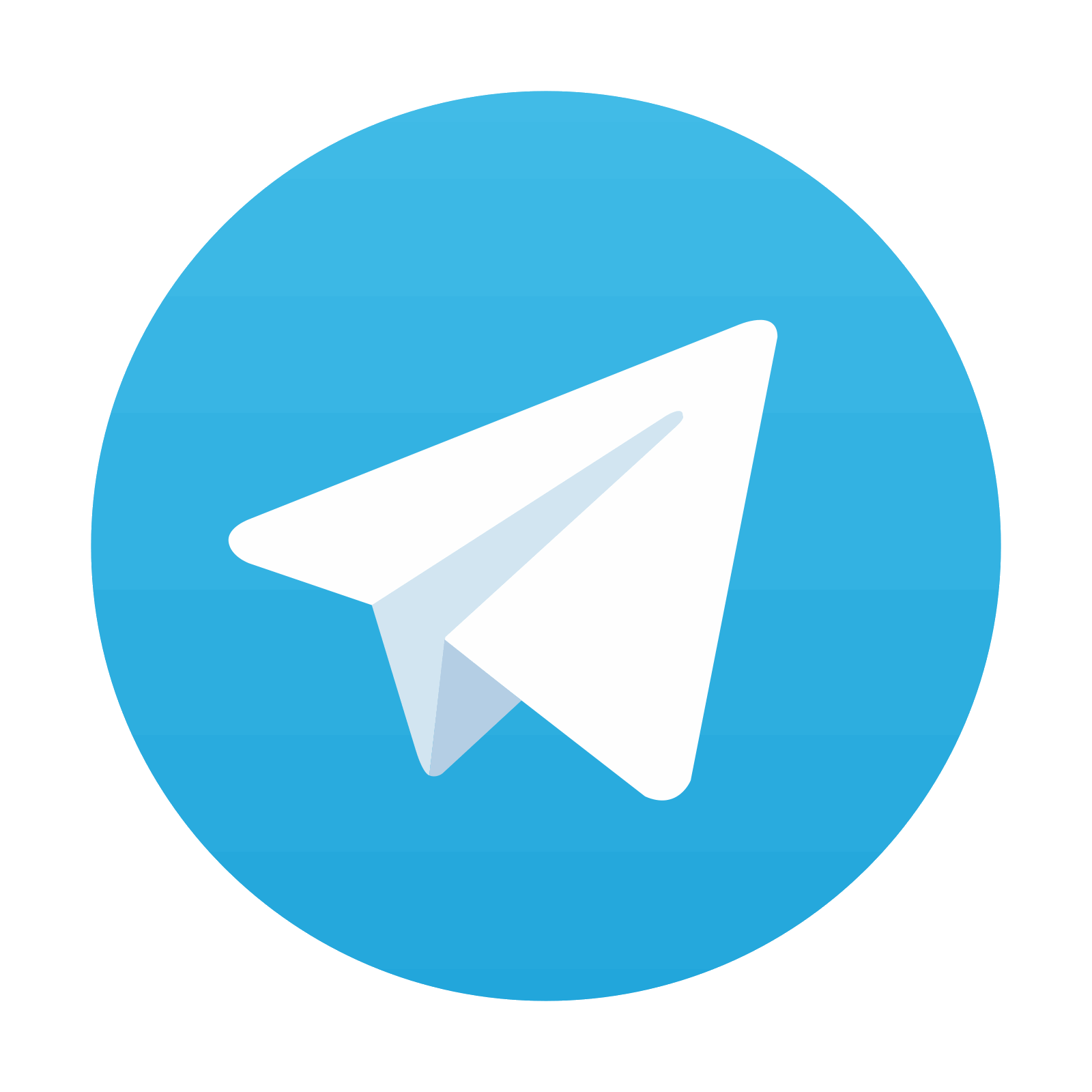
Stay updated, free articles. Join our Telegram channel
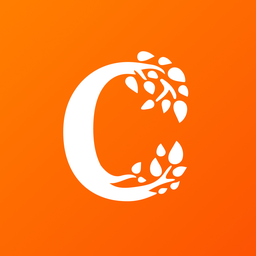
Full access? Get Clinical Tree
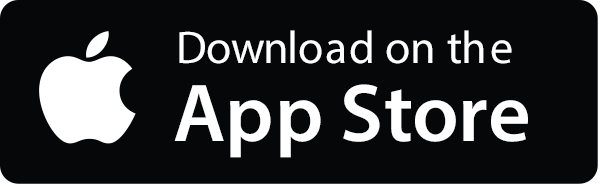
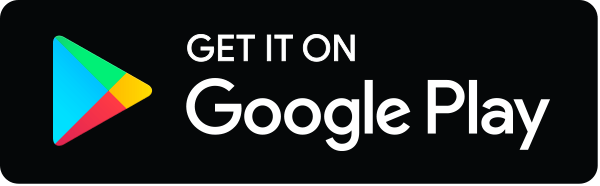
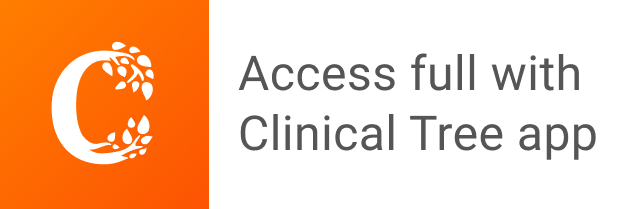