Fig. 5.1
Schematics of a cyclotron. A and B dees with vacuum, D deflector, S ion source, V alternating voltage, W window
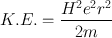
(5.1)
The beam energy may range from a few keV to several billion electron volts (BeV or GeV) depending on the design of the cyclotron. Charged particles such as protons, deuterons, α-particles, H− etc. can be accelerated in a cyclotron. In some special cyclotrons, heavy ions like 3 2S are also accelerated.
There are several features that distinctively characterize positive ion or negative ion cyclotrons. Negative ion cyclotrons need to be run under relatively higher vacuum than positive ion cyclotrons(~ 10−7 Torr vs 10−5 Torr), because H− can lose electrons by encounters with any molecule during acceleration. The beam extraction efficiency in positive ion cyclotrons is ~ 80 % and the remaining 20 % is lost by interaction with the cyclotron housing inducing radioactivity. This warrants more shielding around these cyclotrons. In contrast, since negative ions are extracted with ~ 100 % efficiency and do not interact with the nucleus of any nuclide, the cyclotron housing is not activated, thus requiring less shielding. Targets can be bombarded internally at any radius of the positive ion cyclotrons for radionuclide production , whereas this is not possible in negative ion cyclotrons for lack of nuclear interaction of negative ions with target nuclei. In latter cyclotrons, the particle beam is intercepted with a thin carbon foil (~ 5 µm) toward the exit of the beam to strip two electrons from H− forming an external proton (positive) beam to cause nuclear reactions in target nuclei. In positive ion cyclotrons, a deflector routes the particle beam outside for external irradiation of targets. A unique advantage of negative cyclotrons is that the particle beam can be split into two beams by placing one carbon stripping foil to cover only a part of the beam area and another foil at some distance away covering the remaining area. Thus, two same targets or two different targets can be irradiated simultaneously with the two beams to produce radionuclides .
Medical cyclotrons are compact negative ion cyclotrons that are commonly used for production of short-lived radionuclides such as 18F , 11C , 13N , 15O , and so on used in positron emission tomography (PET) imaging. These nuclides are produced with low energy particles and hence the small size of the cyclotron that can be installed in a small room. In contrast, several medically-useful radionuclides such as 111In, 67Ga , etc. require high energy particles and so large cyclotrons, normally positive ion cyclotrons, are used for their production. The typical energy of the medical cyclotrons ranges between 3–18 MeV.
Some medical cyclotrons have provisions for acceleration of deuteron and H−interchangeably. Whereas high energy cyclotrons are shielded mostly by thick concrete walls, medical cyclotrons are self-shielded with lead blocks for reasons of compact nature. Four lead block quadrants are mounted on casters that wheel them in and out for closing and opening for easy access to service the cyclotron. Siemens, GE Healthcare, IBA, Best Cyclotrons, and Advanced Cyclotron Systems are the major manufacturers of medical cyclotrons in the USA.
When targets of stable elements are irradiated by placing them in the external beam of the accelerated particles or in the internal beam at a given radius inside a cyclotron, the accelerated particles interact with the target nuclei, and nuclear reactions take place. In a nuclear reaction, the incident particle may leave the nucleus after interaction with a nucleon, leaving some of its energy in it, or it may be completely absorbed by the nucleus, depending on the energy of the incident particle. In either case, a nucleus with excitation energy is formed and the excitation energy is disposed of by the emission of nucleons (i.e., protons and neutrons). Particle emission is followed by γ-ray emission when the former is no longer energetically feasible. Depending on the energy deposited by the incident particle, several nucleons are emitted randomly from the irradiated target nucleus, leading to the formation of different nuclides. As the energy of the irradiating particle is increased, more nucleons are emitted, and therefore a wider variety of nuclides is produced.
An example of a typical cyclotron-produced radionuclide is 111In, which is produced by irradiating 111Cd with 12-MeV protons in a cyclotron. The nuclear reaction is written as follows:

where 111Cd is the target nuclide, the proton p is the irradiating particle, the neutron n is the emitted particle, and 111In is the product radionuclide. In this case, a second nucleon may not be emitted, because there is not enough energy left after the emission of the first neutron. The excitation energy that is not sufficient to emit any more nucleons will be dissipated by γ-ray emission.
As can be understood, radionuclides produced with atomic numbers different from those of the target isotopes do not contain any stable (“cold,” or “carrier ”) isotope detectable by ordinary analytical methods, and such preparations are called carrier-free . In practice, however, it is impossible to have these preparations without the presence of any stable isotopes . Another term for these preparations is no-carrier-added (NCA) , meaning that no stable isotope has been added purposely to the preparations.
The target material for irradiation must be pure and preferably monoisotopic or at least enriched isotopically to avoid the production of extraneous radionuclides. Because various isotopes of different elements may be produced in a target, it is necessary to isolate isotopes of a single element; this can be accomplished by appropriate chemical methods such as solvent extraction, precipitation, ion exchange , and distillation. Cyclotron-produced radionuclides are usually proton-rich and therefore decay by β +-emission or electron capture.
Reactor-Produced Radionuclides
A variety of radionuclides is produced in nuclear reactors . A nuclear reactor is constructed with fuel rods made of fissile materials such as enriched 235U and 239Pu. These fuel nuclei undergo spontaneous fission with extremely low probability. Fission is defined as the breakup of a heavy nucleus into two fragments of approximately equal mass, accompanied by the emission of two to three neutrons with mean energies of about 1.5 MeV. In each fission, there is a concomitant energy release of ~ 200 MeV that appears as heat and is usually removed by heat exchangers to produce electricity in the nuclear power plant .
Neutrons emitted in each fission can cause further fission of other fissionable nuclei in the fuel rod, provided the right conditions exist . This obviously will initiate a chain reaction, ultimately leading to a possible meltdown of the reactor core. This chain reaction must be controlled, which is in part accomplished by the proper size, shape, and mass of the fuel material and other complicated and ingenious engineering techniques . To maintain a self-sustained chain reaction, only one fission neutron is needed and excess neutrons (more than one) are removed by cadmium rods, called control rods , which are positioned in the reactor core (cadmium has a high probability of absorbing a thermal neutron). Babcox & Wilcox and Westinghouse are the major manufacturers of nuclear reactors in the USA.
The fuel rods of fissile materials are interspersed in the reactor core with spaces in between. Neutrons emitted with a mean energy of 1.5 MeV from the surface of the fuel rod have a low probability of interacting with other nuclei and therefore do not serve any useful purpose. It has been found, however, that neutrons with thermal energy (0.025 eV) interact with many other stable nuclei efficiently, producing various radionuclides. To make the high-energy neutrons, or so-called fast neutrons , more useful, they are thermalized or slowed down by interaction with low molecular weight materials, such as water, heavy water (D2O), beryllium, and graphite (C), which are distributed in the spaces between the fuel rods. These materials are called moderators . The flux, or intensity, of the thermal neutrons so obtained ranges from 1011 to 1014 neutrons/cm2 ·s, and they are useful in the production of many radionuclides . When a target element is inserted in the reactor core, a thermal neutron will interact with the target nucleus, with a definite probability of producing another nuclide . The probability of formation of a radionuclide by thermal neutrons varies from element to element.
In the reactor, two types of interaction with thermal neutrons occur to produce various radionuclides: fission of heavy elements and neutron capture or (n, γ) reaction. These two nuclear reactions are described next.
Fission or (n, f) Reaction
When a target of heavy elements is inserted in the reactor core, heavy nuclei absorb thermal neutrons and undergo fission . Fissionable heavy elements are 235U , 239Pu, 237Np, 23 3U, 232Th, and many others having atomic numbers greater than 92. Fission of heavy elements may also be induced in a cyclotron by irradiation with high-energy charged particles, but fission probability depends on the type and energy of the irradiating particle. Nuclides produced by fission may range in atomic number from about 28 to nearly 65 . These isotopes of different elements are separated by appropriate chemical procedures that involve precipitation, solvent extraction, ion exchange , chromatography, and distillation. The fission radionuclides are normally carrier-free or NCA , and therefore radionuclides of high specific activity are available from fission. The fission products are usually neutron rich and decay by β −-emission.
Many clinically useful radionuclides such as 131I, 99Mo , 133Xe, and 137Cs are produced by fission of 235U . An example of thermal fission of 23 5U follows, showing a few representative radionuclides:







Many other nuclides besides those mentioned in the example are also produced. For 99Mo production, high enriched uranium (HEU) is commonly used for irradiation, which contains more than 90 % 23 5U in the target. The irradiation is carried out for 5–10 days. Now investigators are attempting to use low enriched uranium (LEU) target that contains less than 20 % 23 5U. This technique requires a longer irradiation for production of sufficient 99Mo and is less expensive. However in view of the current problem in sufficient supply of 99Mo, this technique has drawn considerable interest in nuclear medicine community.
Neutron Capture or (n,γ) Reaction
In neutron capture reaction , the target nucleus captures one thermal neutron and emits γ-rays to produce an isotope of the same element. The radionuclide so produced is therefore not carrier-free , and its specific activity is relatively low. This reaction takes place in almost all elements with varying probability. Some examples of neutron capture reactions are 98Mo(n, γ)99Mo, 196Hg(n, γ)197Hg, and 50Cr(n, γ)51Cr. Molybdenum-99 so produced is called the irradiated molybdenum as opposed to the fission molybdenum described earlier. This method is commonly used in the analysis of trace elements in various samples.
The method of production and various characteristics of radionuclides commonly used in nuclear medicine are presented in Table 5.1.
Table 5.1
Characteristics of commonly used radionuclides
Nuclide | Physical half-life | Mode of delay (%) | γ-ray energy* (MeV) | γ-ray abundance (%) | Common production method |
---|---|---|---|---|---|
![]() | 12.3 year | β − (100) | – | – | 6Li(n, α)3H |
![]() | 20.4 min | β + (100) | 0.511 | 200 | 10B(d, n)11C |
(Annihilation) | 14N(p, α)11C | ||||
![]() | 10 min | β + (100) | 0.511 | 200 | 12C(d, n)13N |
(Annihilation) | 16O(p, α)13N | ||||
13C(p, n)13N | |||||
![]() | 5730 year | β − (100) | – | – | 14N(n, p)14C |
![]() | 2 min | β + (100) | 0.511 | 200 | 14N(d, n)15O |
(Annihilation) | 15N(p, n)15O | ||||
![]() | 110 min | β + (97) | 0.511 | 194 | 18O(p, n)18F |
EC (3) | (Annihilation) | ||||
![]() | 14.3 days | β − (100) | – | – | 32S(n, p)32P |
![]() | 271 days | EC (100) | 0.014 | 9 | 56Fe(d, n)57Co |
0.122 | 86 | ||||
0.136 | 11 | ||||
![]() | 71 days | β + (14.9) | 0.811 | 99.5 | 55Mn(α, n)58Co |
EC (85.1) | |||||
![]() | 45 days | β − (100) | 1.099 | 56 | 58Fe(n, γ)59Fe |
1.292 | 43 | ||||
![]() | 5.2 year | β − (100) | 1.173 | 100 | 59Co(n, γ)60Co |
1.332 | 100 | ||||
![]() | 9.3 h | β + (8) | 0.420 | 25 | 63Cu(p, 2n)62Zn |
EC (92) | 0.511 | 31 | |||
0.548 | 15 | ||||
0.597 | 26 | ||||
![]() | 9.7 min | β + (97) | 0.511 | 194 | 62Ni(p, n)62Cu |
EC (3)
![]() Stay updated, free articles. Join our Telegram channel![]() Full access? Get Clinical Tree![]() ![]() ![]() |