Fig. 2.1
Patient flow through the facility, highlighting hot, cold and shielded stages, and colour coded in proportion to the hazard
Consideration of the following points is recommended to minimise staff exposure and the cost of shielding materials:
- 1.
Maximise the distance between hot patients and staff/members of the public.
- 2.
Eliminate lines of sight between uptake bays and the operator console of the scanner (CCTV and intercoms can be built in cost-effectively at the design stage).
- 3.
Use suitable local shielding for stock vials, syringes, waste, etc.
- 4.
Consider the use of remote injectors and/or dispensers.
- 5.
Consider the handling of QC sources.
The basic hazards to consider are:
External dose rate hazard
Contamination
Emergency situations, e.g. a dropped vial
Standard radiation protection principles should be applied, i.e.:
Distance
Shielding
Time
The inverse square law is very powerful and can dramatically reduce the level of shielding required.
Figure 2.2 shows a facility where there are no direct lines of sight between the rest bays and the control room where staff will spend most of their working time, i.e. there is always a barrier (which may be a wall) between the patient and the areas where staff spend significant periods of time. The preparation room is close to the rest bays and under supervision by staff in the control room and/or the office. The distance from the rest bays to high occupancy areas is maximised which will reduce the required shielding and minimise building costs. The hot WC(s) is/are close to the rest bays.
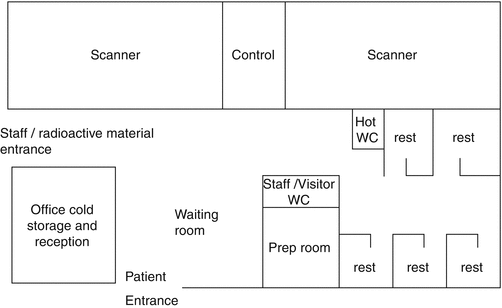
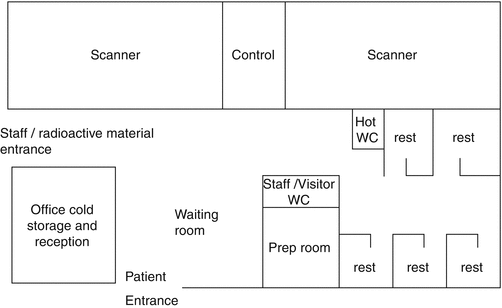
Fig. 2.2
An example of a well-designed facility
For maximum throughput with a single scanner, a minimum of three uptake bays are required. New scanners could increase throughput (or use reduced administered activity) which could impact on this choice.
Figure 2.3 shows a more challenging layout adopted on a number of mobile scanners, where staff in the control room are irradiated by patients in the uptake room and the scanner. The shielding required in this situation is much higher than for the facility in Fig. 2.2.
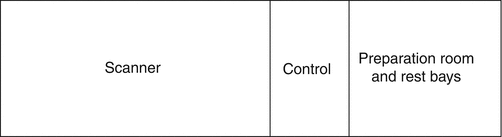
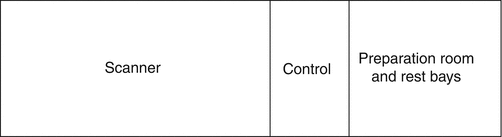
Fig. 2.3
Common layout for a mobile PET/CT scanner
2.2.1 Shielding Calculations
Once the layout is established, shielding calculations are required to specify the thickness of barriers around the facility. The choice of building/shielding material may be clear – but should be established.
Common bricks and blocks may be the cheapest materials to use, although some contractors prefer poured concrete. Lead may be the only solution particularly for mobile vans. As some barriers may have a considerable thickness (up to 300 mm poured concrete), this may impact on the design/space requirements. The attenuation properties of common shielding materials can be found in AAPM Report 108 and the BIR report on shielding in diagnostic x-ray [5, 6]. A simple methodology using limiting tenth value layers (TVLs) is used below. The values for lead and concrete are shown in Table 2.1.
Table 2.1
Limiting TVLs for lead and concrete
Material | Limiting TVL (mm) |
---|---|
Lead | 15 |
Concrete (density 2350 kg/m3) | 150 |
The TVL for other materials such as concrete blocks can be extrapolated using the ratio of physical density, e.g. 176 mm for concrete blocks with density 2000 kg/m3.
Typical transmission factors for common materials are shown in Table 2.2.
Table 2.2
Typical transmission factors for building materials
Thickness and material | Transmission F-18 |
---|---|
2.24 mm Pb | 0.738 |
4½″ brick | 0.03 |
9″ brick | 0.09 |
100 mm breeze block | 0.66 |
100 mm solid concrete block | 0.27 |
200 mm poured concrete | 0.046 |
The air kerma rate immediately postinjection of 370 MBq of F-18 FDG has been reported to be 45 μGyh−1 at 1 m [9]. There is some self-absorption within the patient of over 30 % from the air kerma rate quoted [1]. Table 2.3 shows the instantaneous dose rates through typical barriers and shielding materials in diagnostic facilities.
Table 2.3
Instantaneous dose rate at 1 m from an injected patient through typical barriers
Thickness and material | Instantaneous dose rate postinjection (μGy/h) |
---|---|
2.24 mm Pb | 33.2 |
4½″ brick | 13.7 |
9″ brick | 4.1 |
100 mm breeze block | 29.8 |
100 mm solid concrete block | 12.2 |
200 mm poured concrete | 2.1 |
It should be noted that for planning purposes, a lower value is used to include a correction for radioactive decay and for excretion during the procedure.
The following examples of layouts and shielding calculations will concentrate on F-18-FDG scanning assuming an administration of 370 MBq, a rest phase of 1 h and a scan time of 20 min.
The following should be established to enable the calculations to be performed:
- 1.
Workload – this will dictate the time a hazard is present in a particular area, e.g. uptake bays, scanner and discharge area.
At worst all uptake bays and the scanner might be occupied 100 % of the time.
- 2.
Dose constraint – this is typically 0.3 mSv per annum to members of the public and 1 mSv to staff. Note it may be difficult to achieve 1 mSv to the staff in a mobile design [10]. It should be noted that the staff will also receive dose from their contact with patients during injection, set-up and discharge. Total dose per annum for a high throughput of patients can approach 6 mSv, so it is important to minimise the dose that the staff receive through the facility design.
- 3.
Calculation points – areas where staff or members of the public may spend time or become close to patients:
Control room
Offices
Rest rooms
Areas above or below the facility
Corridors
Clinic rooms
Occupancy in these areas will need to be estimated. Some common occupancy factors are described in the BIR publication [5] with a range quoted to enable local knowledge of the use of the area to be applied. The minimum occupancy that is recommended to be applied is 5 % for car parks and other transiently occupied areas. Corridors may be between 10 and 20 %, but if it is known that a corridor is very rarely used, a factor of 5 % could be applied. Potential changes in the use of such areas in the future needs to be considered particularly if a low occupancy factor is applied. The distance between the source and the calculation points should be assessed.
The following dose rates can be used for planning purposes. They include integrating the initial instantaneous dose rate over the 1 h uptake phase and include a factor for evacuation of the bladder and decay over a 20 min scan [5]:
37 μGyh−1 at 1 m for the uptake phase
24 μGyh−1 at 1 m for the scan phase
The values are slightly more conservative than those quoted by the AAPM [6].
The barrier thickness is calculated by considering the critical points around a room such as the uptake bay. If the adjacent room is an office which is occupied 100 % of the time, and the critical point, e.g. the office chair, is 2 m from the centre of the rest bed:
Using this distance from the patient – 2 m.
Air kerma rate at 1 m from the patient is 37 μGy/h.
The kerma rate corrected for distance is 9.25 μGy/h.
The dose per annum (over 2000 h) is 18.5 mSv.
The chosen constraint is 0.3 mSv.
The attenuation factor (AF) or transmission required is no more than 0.3/18.5 or 0.02.
The number of TVLs to attenuate to this factor is log10 (1/AF).
Therefore, 1.79 TVLs are required, i.e. 270 mm concrete or 30 mm lead.
This can be repeated many times over to look at all critical points around the facility. Some centres choose to look at the worst-case point and apply that barrier thickness to the whole room to minimise the chance of mistakes during construction.
However, individuals are likely to be irradiated from more than one source, and the doses from all should be considered and summed [5]. If the approach above is used, the dose constraint could be divided between all sources, or an iterative approach using different wall thicknesses to share the dose burden more equally can be used. A number of authors have described different approaches [11–13].
The resultant required wall thickness can vary, but 300 mm concrete will almost always be adequate. This is to protect persons around the facility and will normally only be required to be a height of 2200 mm above finished floor level. Areas above and below may need some consideration.
CT scatter requires shielding to the underside of the soffit [5]. This might be most cost-effectively achieved by fitting a minimum of 1.3 mm lead above the walls to the soffit. The walls below this height will normally be thicker than this to protect from the gamma rays. Consideration needs to be given to air conditioning and other service access into the room. The penetrations can be large and often in the worst place from a radiation protection perspective, e.g. over the doors, which is often the place where scatter is highest.
2.2.2 Staff Exposure
Staff exposure is potentially high as can be seen by the shielding calculations above. The member of the public dose limit is exceeded with 12 h at a metre from a patient during the uptake phase. Whole-body personal monitoring is recommended.
Finger dose can be exceptionally high with 500 mSv being reached within 3 h at a distance of 100 mm from a stock vial containing 10 GBq of activity. The inverse square law breaks down at closer distances, but it is clear that shielding and good technique are required. Finger dose monitoring is recommended for all staff manipulating radioactive sources in PET.
Eye doses may need to be considered in the light of the new dose limits [14] if whole-body doses are very high, but recent publications suggest that with adequate controls, dose limits should not be approached. However, radiation dose management in all hybrid imaging especially PET/CT can be a concern [15]. Direct reading dosemeters can be very useful devices to look at daily dose, to set alarms for staff who stand close to patients and can be used as an aid to audit.
2.2.3 Optimisation: Fixtures, Fittings and Accessories
Optimisation in terms of radiation protection is about keeping doses as low as reasonably achievable. The external dose rate hazard and the contamination risk both need to be considered. For the external dose rate hazard, the basic principles of operation in radiation protection apply:
Distance
Shielding
Time
Distance is a powerful measure as the inverse square law comes into play. The basic design and layout can be used to maximise the distance between patients and staff, but there are times when staff need to be close to sources and the patient. The greatest hazard is from the stock vial which will need to be unpacked and measured. This could typically hold 10–80 GBq of activity. Simple handling tools can be used to maximise the distance between finger tips and the source (Fig. 2.4). Long-handled forceps and other devices commonly used in nuclear medicine or radiotherapy can also be used.
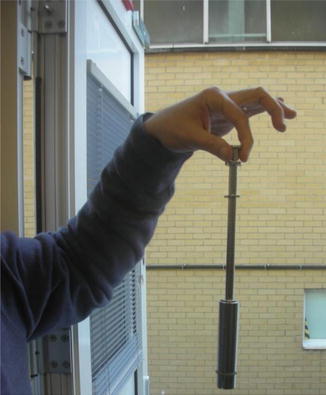
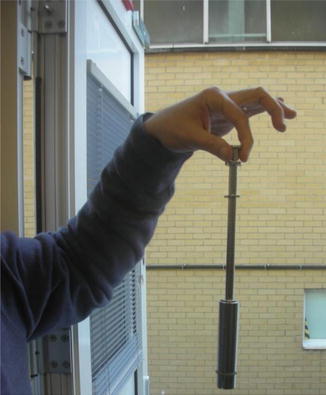
Fig. 2.4
Long-handled tool for handling stock vial
The vial is generally placed into a dispensing unit which incorporates thick shielding (Fig. 2.5) and is designed to minimise exposure.
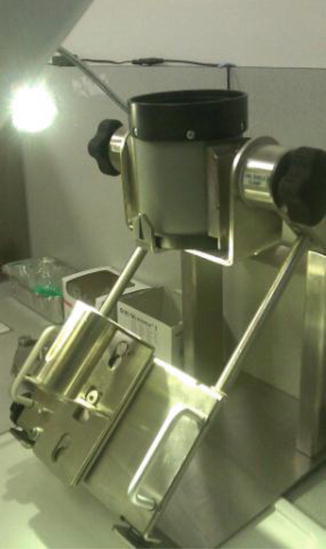
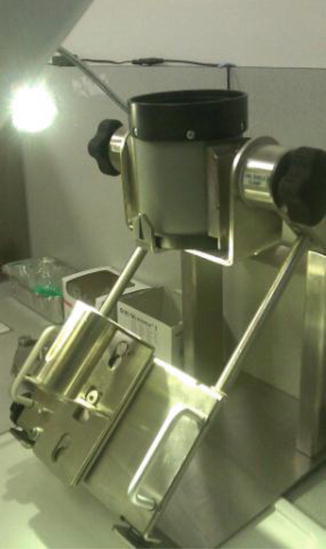
Fig. 2.5
Heavily shielded manual dispensing unit to hold the stock vial
Automatic dispensing units are also commercially available and might be worth considering in high-throughput units. It is common practice to sink the dose calibrator into the preparation bench and to have a route to drop waste into a shielded waste bin. Bench top shields are recommended to protect the body and the eyes (Fig. 2.6).
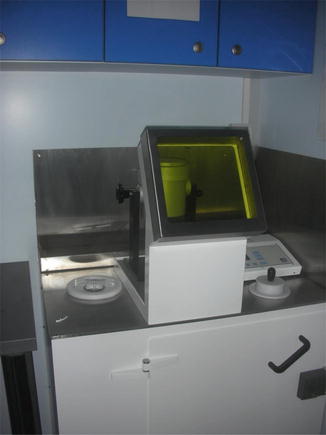
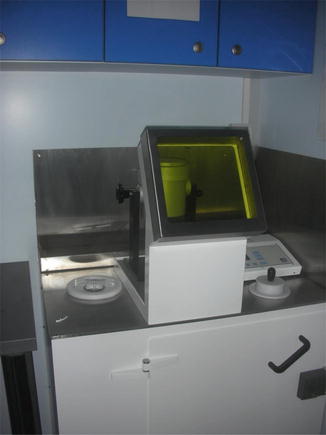
Fig. 2.6
Dispensing unit with bench top shield, sunken calibrator and waste route into shielded cupboard
All this local shielding is thicker than conventionally used in nuclear medicine. The weight may also need to be considered. Entry and exit portal monitors need careful consideration to eliminate background and to ensure contamination is not spread.
Plastic scintillators are used to detect beta emissions from the radionuclide although the gamma emissions are sufficient that some conventional sensitive scintillators may be adequate for contamination monitoring [16]. Storage and handling of sources used for quality control need to be considered. Sources should always be handled at the opposite end from where the activity is sited. Once doses have been dispensed, a syringe shield is required and the injection should be carried in a shielded box (Fig. 2.7).
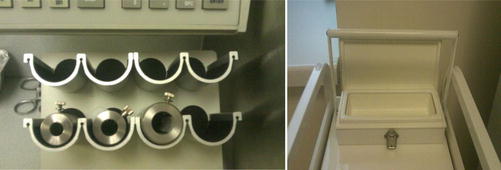
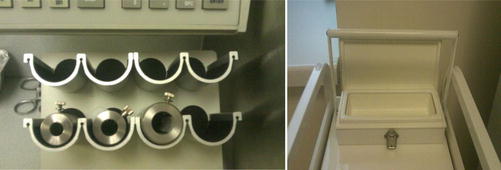
Fig. 2.7
Syringe shields and carrying boxes for individual injections
There has been some debate as to whether Perspex or tungsten syringe shields reduce finger dose more effectively, but it is generally accepted that tungsten is more effective [17]. All syringe shields are bigger and more bulky than conventional shields, and staff should be initially trained to manipulate the radionuclide with inactive solutions. All surfaces must be easy to clean and decontaminate, flooring should be smooth without joints and cover up the wall and there needs to be space for decontamination kits and materials.
Risk assessments are essential to assess the level of protection required. High workload departments may need more automated accessories to keep doses low.
Contingency plans must be prepared for all obvious problems – fire, flood, theft and contamination. The action to be taken in the event of a stock vial being broken or a single injection being dropped should be known and understood by all staff involved. Contingency plans should be practised. All staff should be experienced in clearing spills, but for training purposes, inactive materials should be used.
2.3 Staff Dose and Optimisation
Once the service is operational, further optimisation of doses is likely to be possible as staff become familiar with processes and local practices.
If the total whole-body dose results for all staff are plotted over time against the number of patients through the system graphs, such in Fig. 2.8 might be observed. This shows that as the workload increases, so does dose efficiency which plateaus out eventually.
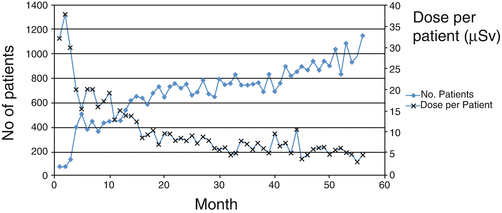
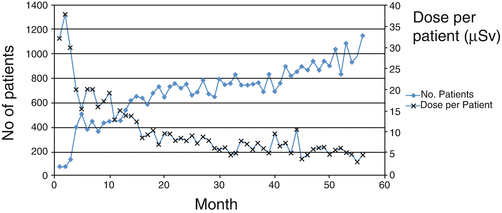
Fig. 2.8
Staff dose over time against increasing workload (Courtesy of InHealth Ltd)
The use of electronic personal dosemeters is advocated to monitor and help minimise dose for the whole procedure and individual parts of the procedure. A number of studies have been published showing the average dose per patient [18–22]. For a 370 MBq injection, the total dose per patient has been reported to be between 3.3 and 9.2 μSv.
Careful measurement of the doses recorded on electronic personal dosemeters can show the dose received during each stage of the process [22] as shown in Fig. 2.9.
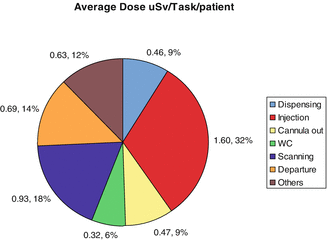
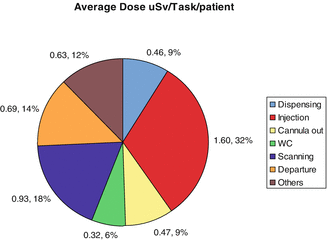
Fig. 2.9
Average dose for each part of the procedure (Republished with permission of British Institute of Radiology, from Peet et al. [22])
Review of doses for individual members of staff can show that the doses vary by up to a factor of 5, giving further opportunity for optimisation and staff training. The opportunity to reduce these doses using automatic dispensers and injectors has been mooted [4]. There is some published data to suggest they can be successful, but it must be remembered they are in no way a substitute for excellent radiation protection in practice. Another tool advocated is to show areas where doses might be high within a facility – highlighting hot spots and the safest places to spend time as shown in Fig. 2.10 [22].
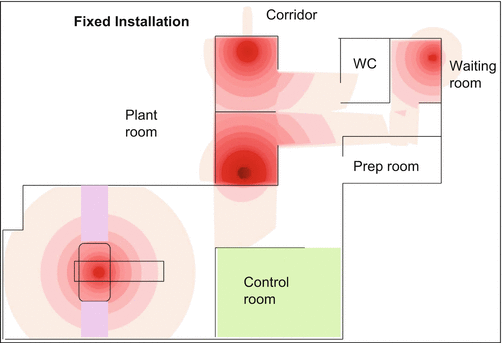
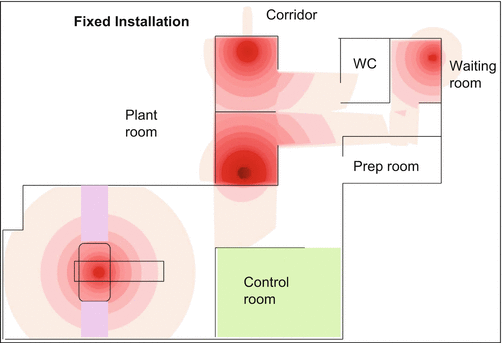
Fig. 2.10
Instantaneous dose rates plotted through the facility (Republished with permission of British Institute of Radiology, from Peet et al. [22])
It should also be noted that optimisation is an ongoing process. Success in reducing doses and keeping them at that level requires constant vigilance. The modality whilst having exciting clinical potential needs particular care around staff and public safety – finger doses and whole-body doses can be high and could without care approach dose limits.
2.4 CT Technology
2.4.1 Basic Technology
A CT scanner consists of a rotating gantry, a couch and an operator’s console. The gantry, within an external housing, consists of an x-ray tube diametrically opposite to an arc of detectors, usually described as a detector row or rows. The whole construction rotates around a central point in space (the isocentre).
The patient lies on the couch whilst the x-ray tube and detectors are rotated around the patient, the x-ray source is activated for a scan and the detectors record the transmission of the x-ray beam through the patient. During this process, the couch can either be stationary, and the x-rays are switched off whilst the couch moves to the next position; this is called axial scanning. Alternatively the couch can be set to move continuously along the patient long axis, usually called the z-axis (Fig. 2.11), and this is called helical or spiral scanning.
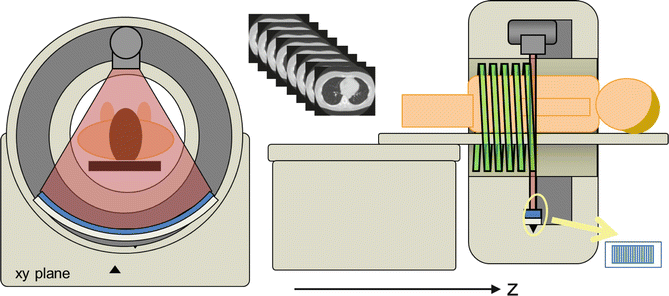
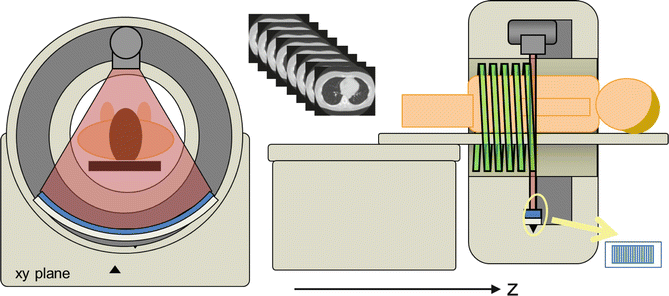
Fig. 2.11
Cross-sectional and lateral view of the CT scanner, with resultant images (Courtesy ImPACT)
The transmission data from the x-ray beam, attenuated by the patient, is collected by the detectors from many angles around the patient, and using either computerised ‘back projection’ or ‘iterative’ processes, a cross-sectional image of the patient is reconstructed.
The cross-sectional image presents maps of the x-ray attenuation properties of the tissues and organs. This can be used in PET/CT scanning to correct the PET image for the attenuation of the 511 keV gamma ray – known as attenuation scanning. This is in addition to the capability for anatomical localisation, as well as fully diagnostic anatomical information which can be acquired with or without contrast injection.
The exposure factors required for an accurate correction of attenuation are generally lower than those required for full diagnostic scanning where a less noisy image might be required. The dose the patient receives is related to these exposure factors, and this section describes the dose indices in common usage in CT and their relationship to effective dose (E).
2.4.2 Technology Developments
CT scanning has undergone many design changes since its inception. The introduction in 1985 of slip rings to transfer power and data, subsequently enabling helical scanning, could be regarded as a significant milestone and perhaps signals the design of modern scanners. In addition, since then, there have been numerous significant technological advances, and some of these need to be outlined to gain an understanding of the current dose indices.
It is not commonly remembered that the very first CT scanners in 1972 were dual-slice scanners, but this approach was very quickly dropped with a change in gantry design, and the single-slice design continued until 1991. Since then, over a period of 15 years, rapid developments in scanner technology have resulted in the transition from dual slice through 8, 16 and 64 ‘slice’ and, in recent years, 320 detector row scanners.
Scanners are usually discussed, and sold, with respect to the competitive number of ‘slices’ that can be imaged in a single rotation. However, this can be extremely misleading. Any particular scanner model may reconstruct fewer (on older scanners) or more (on newer scanners) image slices per rotation than the number of detector rows. For example, an old 16-slice scanner may have 24 detector rows – the limitation at the time of their introduction being in terms of processing, cost of construction of thin detector elements and reconstruction capacity. Conversely a newer ‘64-slice’ scanner may have 32 detector rows, a ‘640 slice’ may have 320 rows or a ‘512 slice’ 256 rows. This greater slice capability at a finer spacing than the actual detector rows is due to modern techniques in reconstruction and can be regarded as overlapping image slices. In terms of speed of coverage, therefore it is far more meaningful to categorise a scanner by the number of detector rows and the length of coverage along the patient axis by the whole detector bank, with consideration being given to the number of image slices acquired simultaneously (Fig. 2.12).
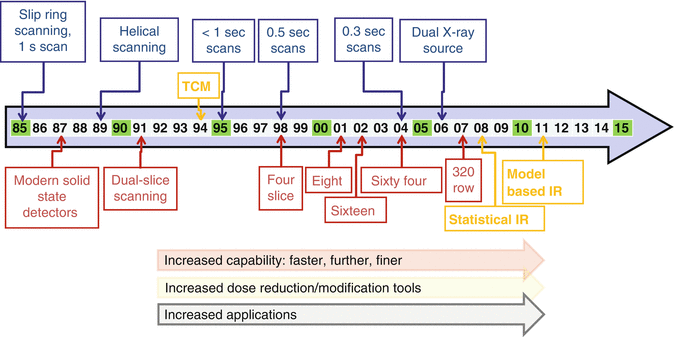
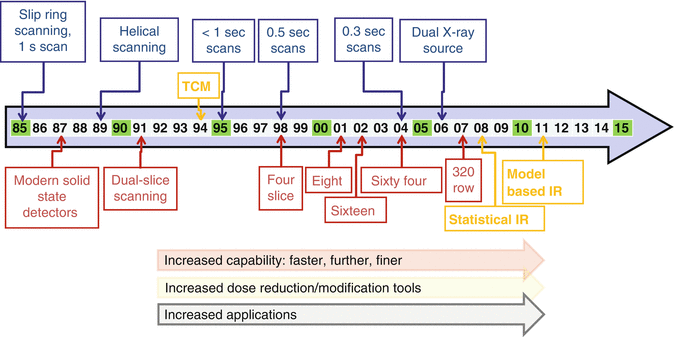
Fig. 2.12
CT technological advances 1985–2014
The time taken to undertake any particular examination scan is now shorter due to faster tube rotation times, longer detector arrays and more detector rows along the z-axis. Tube rotation times per revolution have been reduced to less than 0.5 s (of the order of 0.3 s to enable cardiac imaging). This together with the greater number of detector rows, and helical scanning, removes the need for breath-hold imaging in many circumstances. A large volume of the patient can therefore be scanned in a relatively short period of time (e.g. 20 s for the chest abdomen and pelvis). Radiation exposure must become a consideration both in terms of equipment design and operation of the scanner.
Image reconstruction has progressed from analytical filtered back projection methods to statistical iterative reconstruction techniques. On most modern scanners, both approaches are available. By the efficient use of the x-ray attenuation information, iterative techniques reduce image noise. Whilst this gives improved image quality, it also alternatively presents the operator an opportunity to lower the tube current (thus reducing the radiation dose), in order to restore the noise to the original level which had previously been accepted as suitable. A good description of CT technology and its evolution can be found in Kalendar’s book [23].
2.4.3 Factors Affecting Dose in CT Scanning
The key parameters that affect patient (organ) dose are those that influence the photons delivered from the x-ray tube. Primarily therefore this is the tube voltage (kV), the tube current (mA) and the rotation time for one revolution(s).
There are also hardware features which are often built into the scan protocol, such as the x-ray beam-shaping filter which relates to patient body part (head or body) scanned (and sometimes patient size), and the x-ray tube focal spot which may be automatically adjusted according to tube current and imaged slice thickness.
In addition image reconstruction features may indirectly affect the dose. For example, the tube current may be set higher for a thinner reconstructed slice to allow enough photons for the required image quality. Reconstruction algorithms will affect the image noise, which also may require adjustment of the tube current.
The pitch in helical scanning may affect the dose, with a longer pitch reducing the average dose along the patient length. However, often the tube current is changed automatically to compensate.
The total dose imparted to the patient is governed by the site-specific (organ) dose, as well as by the scan length. A relevant aspect for consideration in helical scanning is that the total length of irradiation will be slightly longer than the resultant imaged volume, due to the interpolation of data in order to create planar images. This is of special relevance for shorter scan volumes, especially with wider beam widths, where the proportional increase in dose may be significant or where the end of the imaged volume is near organs of particular concern.
Most of the major manufacturers now have a ‘dynamic collimation’ that automatically, in real time, closes off the beam at the trailing and forward edges of the helical irradiation, i.e. at the beginning and end of the scan run, respectively. This ensures that unnecessary radiation is eliminated whilst keeping the appropriate transmission information in order to reconstruct the first and last images. Wider beam widths have the advantage of less penumbra proportionately and also faster scanner of a volume. Dynamic collimators allow the use of wider beam widths without the penalty of extra irradiation at either end of the scan run.
Automatic exposure control (AEC) functions are available for all scanners. They operate by adjusting the tube current in order to attempt to match the tube x-ray output to the attenuation of the patient – adjusting according to the whole size, the relative rotational dimensions or along the patient long axis.
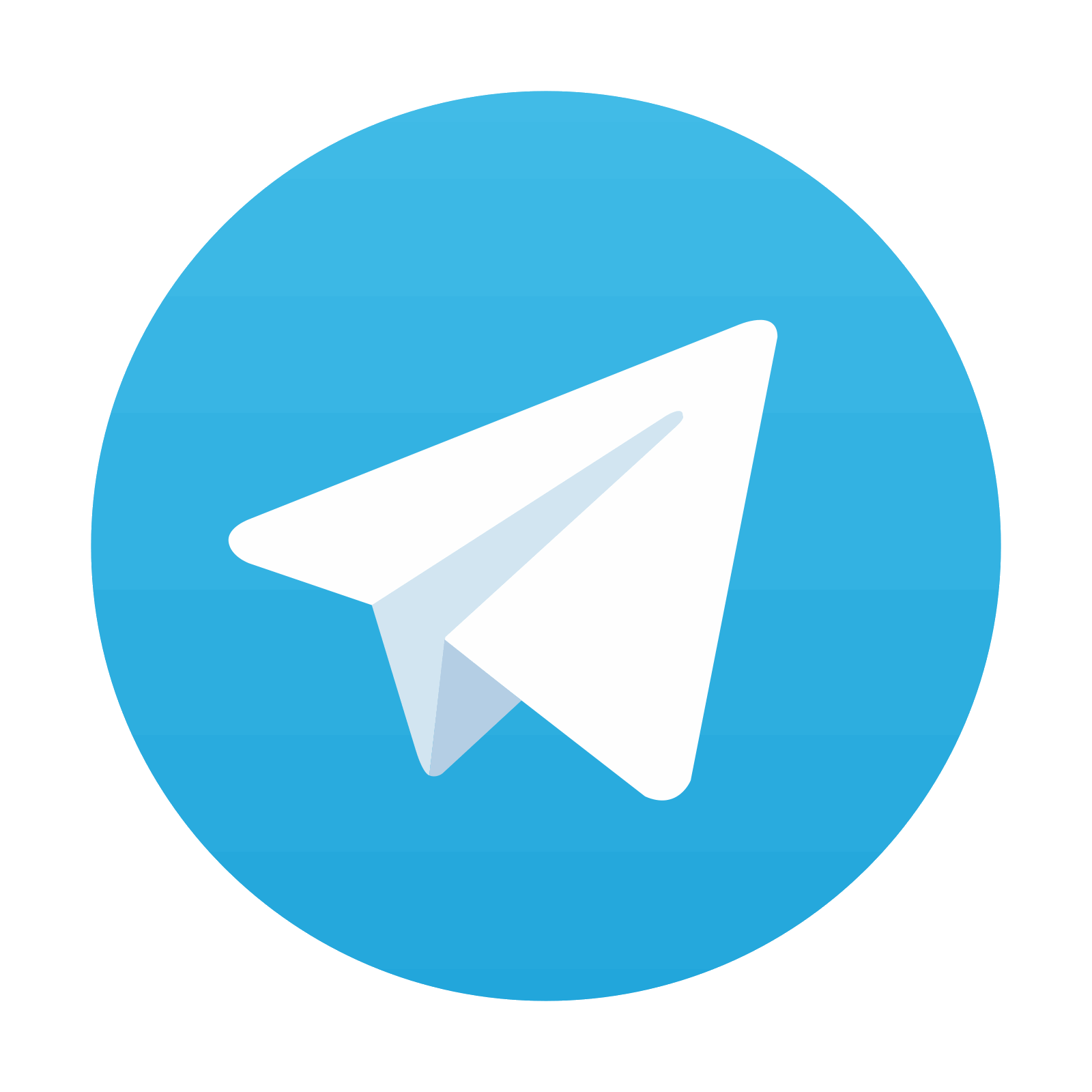
Stay updated, free articles. Join our Telegram channel
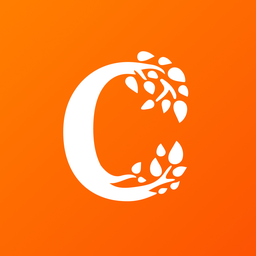
Full access? Get Clinical Tree
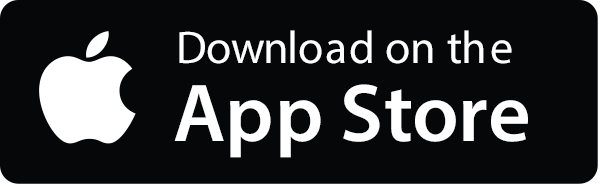
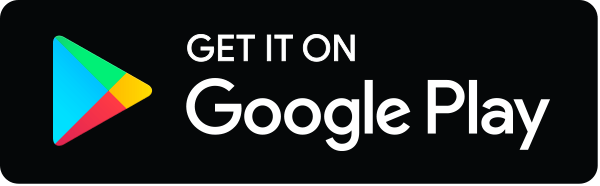