Radiobiology of Charged Particles
Leo Gerweck
Harald Paganetti
ENERGY DEPOSITION AND RELATIVE BIOLOGICAL EFFECTIVENESS
The number of ionized biomolecules produced per unit dose of protons, heavier charged particles and x-rays is similar, but the resulting biological effects substantially differ. As seen in Figure 2.1, the fraction of cells surviving a particular dose of x-irradiation is larger than the fraction of cells surviving the same dose of charged particles. This difference in effectiveness is characterized by the ratio of the dose of 250 kVp x-irradiation to produce a specified effect, to the dose of test irradiation to produce the same effect, and is termed the relative biological effectiveness or RBE. Owing to the large clinical experience with megavoltage x-rays, the most common and preferred reference radiation for treatment planning purposes is megavoltage x-rays or higher-energy photons such as cobalt 60 (60Co). Effect may refer to cell killing, mutation, transformation, that is, carcinogenesis, tissue damage, or other end points. For virtually all types of beams, doses, and end points of primary interest to radiation oncologists, the RBE of charged particles is ≥1.0. Substantial differences in biological effect per unit dose occur not only between megavoltage x-rays and charged particles, but also between different charged particles and the same charged particles at different energies. The change in biological effect per unit dose that occurs secondary to changes in the energy or velocity of charged particles provides important clues to the factors contributing to the increased RBE of these particles.
For monoenergetic beams, the energy transferred to the absorbing medium per unit track length of the particle, or linear energy transfer (LET), increases to a maximum as the velocity of the particles slows near the end of their range and the particles come to rest. The region of maximum energy transfer is termed the Bragg peak. For several biological end points, a characteristic relation is observed between the change in LET and the RBE of a specific particle beam. For protons, the biological effect increases throughout the Bragg peak, reaching a maximum near the distal edge of the peak. As the particles’ velocity continues to decrease and LET increases, the killing efficiency per unit dose decreases.
Figure 2.2 shows the relation between energy-deposition density or LET and biological effect for protons and carbon ions. The LET at which the maximum RBE is observed is particle specific. Carbon exhibits a higher maximum RBE, and the increased RBE manifests over a larger LET range than pertains to protons. Ionization density per unit track length is only an approximate descriptor and determinant of biological effect.
Figure 2.2 shows the relation between energy-deposition density or LET and biological effect for protons and carbon ions. The LET at which the maximum RBE is observed is particle specific. Carbon exhibits a higher maximum RBE, and the increased RBE manifests over a larger LET range than pertains to protons. Ionization density per unit track length is only an approximate descriptor and determinant of biological effect.
The Critical Target for Biological Effects
Insight into the relation between ionization density and biological effect has been achieved by the identification of the critical target for cell killing and its dimensions, relative to the pattern of energy deposition. For mutation induction, carcinogenic transformation, and killing of most cell types, substantial evidence shows that damage to the DNA molecules is the causative lesion. This evidence includes, but is not limited to, the inefficiency of irradiating the cell membrane and cytoplasm versus the cell nucleus; the greater killing efficiency of 3H-labeled biomolecules incorporated into DNA but not RNA, lipid or protein; and the relation between cell killing and chromosome aberrations. Breaks in opposite strands of DNA that are in close proximity give rise to double-strand breaks (DSBs) either directly or during the strand-break repair process, and unrepaired DSBs lead to the dysfunction and loss of genetic material, chromosome aberrations, and cell death. This suggests that charged particles and ionization densities that more efficiently produce DNA DSBs or alternatively produce less repairable DSBs will result in a greater biological effect per unit dose. DSBs may arise from the direct deposition of energy in the DNA or from the ionization of water within a few nanometers of the DNA, that is, within the diffusion distance of the radicals produced by radiation. The passage of densely ionizing tracts of charged particles through cells and consequent damage to DNA can be visualized by staining irradiated cells with fluorescent-labeled antibodies to proteins known to accumulate at the site of and contribute to the repair of DNA DSBs. Figure 2.3 shows the tracks produced by the passage of bismuth ions through a mammalian cell nucleus.1
Table 2.1 shows the approximate number of events in a mammalian cell following low-LET x-irradiation versus high-LET irradiation after a dose of 1 Gy.2,3 Both radiations produce approximately 100,000 ionizations in the nucleus and 1,500 ionizations in DNA, resulting from approximately 1,000 diffuse low-LET tracts and two tracts of densely ionizing α particles. The initial yield of DSBs shows little variation with ionization density, but the number of residual breaks at 8 hours after cellular repair substantially differs. Similarly, the number of initial chromosome breaks as revealed by premature chromosome condensation (PCC) is similar following low- and high-LET radiation, but markedly differs following 8 hours of cellular repair of the DNA damage. The same number of initial DSBs gives rise to a substantially larger number of chromosome aberrations following high-LET versus low-LET irradiation, with consequent effects on cell viability. In addition to increasing the number of residual chromosome
breaks, high-LET radiation also gives rise to changes in the frequency of specific types of aberrations including complex aberrations.4
breaks, high-LET radiation also gives rise to changes in the frequency of specific types of aberrations including complex aberrations.4
TABLE 2.1 AVERAGE YIELD OF DAMAGE IN A SINGLE MAMMALIAN CELL AFTER 1-GY IRRADIATIONa | |||||||||||||||||||||||||||||||||||||||||||||
---|---|---|---|---|---|---|---|---|---|---|---|---|---|---|---|---|---|---|---|---|---|---|---|---|---|---|---|---|---|---|---|---|---|---|---|---|---|---|---|---|---|---|---|---|---|
|
Although particle irradiation yields the same number of DNA DSBs per unit dose as low-LET x-irradiation, the distribution of the DSBs substantially differs between low- and high-LET radiation. The fraction of small DNA fragments of 100 to 2,000 base pairs as a percentage of total fragments produced increases with increasing LET.5 Monte Carlo track structure calculations of high-LET ionization in a solenoidal model of DNA are consistent with these experimental observations.6 For high-LET radiations, the calculated values and experimental evidence indicates that substantial energy transfer occurs through the deposition of relatively large quantities or packets of energy in DNA structures spanning nm. This clustering of energy deposition results in multiple DSBs along with single-strand breaks, DNA crosslinks, base damage and excitation in a nucleosome or larger 25- to 30-nm segments of chromatin.3,5,7 Therefore, the major difference between low- and high-LET radiation or high-energy protons versus protons near their densely ionizing track ends is the deposition of energy in localized regions of the DNA, nucleosome, or chromatin fiber. Although the average macroscopic number of events does not differ, the clustering of strand breaks and associated damage is believed to render the lesions less amenable to repair or competent repair.
Evidence that high-LET radiation produces less repairable DNA DSBs is provided by analysis of the response of mutant cell lines deficient in various DNA DSB repair pathways and their repair-proficient parental cells. Cells deficient in the homologous recombination pathway for DSB repair as well as in the nonhomologous end-joining repair pathway, are more sensitive to both low-LET and high-LET radiation than their repair-competent parental cell line.8 However, in repair-deficient cells, the increased sensitivity to x-rays is more pronounced than the increased sensitivity to high-LET radiation, and the RBE is therefore substantially reduced in DSB repair-deficient cells as compared to DSB repair-proficient cells. DSBs produced by low-LET radiation are more amenable to repair than DSBs produced by high-LET radiation.
To summarize, for the same charged particle, a change in the velocity and LET of the particles gives rise to a change in RBE. Multiply damaged sites within the same locus or small segments of chromatin fiber by high-LET radiation render DSBs that are less amendable to repair. Nevertheless, LET is only an approximate indicator of the relation between the pattern and density of energy deposition and biological effect. At the same LET, different ions exhibit substantially different RBE values for a variety of end points including cell killing, and the LET yielding the maximum RBE decreases with the decreasing Z of the particle. Through the continued detailed analyses of the spatial patterns of energy deposition for various charged particles relative to the dimensions of DNA in its various configurations, a more robust parameter of RBE may be developed. However, as discussed in the section “The Relative Biological Effectiveness of Clinical Spread-out Bragg Peak Beams,” the RBE of a particle is dependent not only on the energy-deposition pattern and nature of the initial lesion produced, but also on the response of the cell to the lesion. This response may vary from cell type to cell type.
The Relative Biological Effectiveness for Apoptosis, Mutation and Transformation
Although the evidence for DNA being the critical site for radiation damage that leads to cell death in most cell types is compelling, for a limited number of cell types primarily of the reticuloendothelial system, a distinct pathway of cell death may be initiated by damage to the cell membrane as well as the DNA. This pathway of cell death, termed apoptosis or programmed cell death, exhibits several molecular and morphologic features that differentiate it from the classic mitosis-linked cell death or necrosis. It manifests commonly, but not exclusively, more rapidly than mitotic cell death, that is, as interphase death before the first mitosis following irradiation. Unlike cell death through necrosis, which increases exponentially with increasing dose of x-irradiation, induction of apoptosis saturates with increasing dose. However, as with cell death through the necrotic pathway, the RBE for apoptosis is LET
dependent. For densely ionizing radiation, for example, 30 to 200 keV per µm iron or carbon beams in the dose range of a few Gy or less, the RBE for apoptosis increases to approximately 3 to 4, independent of p53 gene status.9, 10, 11 The RBE for apoptosis is in the same range as the RBE for cell killing through the chromosome aberration-linked necrotic pathway of cell death.
dependent. For densely ionizing radiation, for example, 30 to 200 keV per µm iron or carbon beams in the dose range of a few Gy or less, the RBE for apoptosis increases to approximately 3 to 4, independent of p53 gene status.9, 10, 11 The RBE for apoptosis is in the same range as the RBE for cell killing through the chromosome aberration-linked necrotic pathway of cell death.
As pertains to cell killing, mutation frequency per unit dose varies with the LET of the charged particle, and the LET at which the maximum RBE is observed is dependent on the atomic number of the charged particle. However, as the expression of mutations is dependent on the cell maintaining its viability, the mutation frequency per exposed cell plateaus with increasing dose and then declines as mutated cells are lethally inactivated. The RBE for mutation at the HGPRT locus has been systematically evaluated in protons at various energies, and found to be approximately equal to the RBE for cell killing.12 These data suggest that per unit cell kill, protons pose no additional risk per surviving cells as compared to low-LET x-rays. In cell killing, the LET for maximum mutation induction is in the range of 25 to 30 keV per µm for protons, and is higher than is observed for other charged particles at the same LET.12 For carbon and neon beams, the maximum RBE values are observed at LET values of approximately 75 and 155 keV per µm.13
The expression of cellular transformation or carcinogenesis following ionizing radiation exhibits many of the same characteristics with respect to the expression of mutations (reviewed in reference14). For both low-LET x-rays and charged particles, transformation increases to a maximum and then decreases with increasing dose as the transformed or potentially transformed cells are killed. However, when evaluated as the number of transformed cells per surviving cell, transformation frequency increases with dose. The LET for maximum transformation efficiency is also particle dependent, being in the range of approximately 30 keV per µm for protons and increasing to higher LET values with the Z of the charged particle. The RBEs for cell killing and transformation are of a similar magnitude.
THE RELATIVE BIOLOGICAL EFFECTIVENESS OF CLINICAL SPREAD-OUT BRAGG PEAK BEAMS
As the dimensions of the Bragg peak are small relative to the typical lesion treated by radiation, and the treatment goal is to maximize dose to the target volume and minimize dose to nontarget tissue, clinically useful particle beams employ spread-out Bragg peak (SOBP) beams. These spread-out beams are generated by employing a monoenergetic beam of sufficient energy and range for coverage of the most distal target volume, together with beams of decreasing energy and decreasing intensity for coverage of the proximal portion of the target volume. The distal most portion of the SOBP therefore predominantly contains Bragg peak high-LET particles, whereas the more proximal portion of the beam increasingly contains higher-energy, lower-LET particles, as illustrated in Figure 2.4.
![]() Figure 2.4 A spread-out Bragg peak is generated by the superimposition of beams of different range and intensity. The broadening of the Bragg peak is achieved by passage of a beam through a rotating wheel of absorbing material containing sectors of different width and thickness. (Adapted with permission from Koehler AM, Preston WM. Protons in radiation therapy. Comparative dose distributions for protons, photons, and electrons. Radiology. 1972;104:191-195.)15 |
RBE may vary not only due to the changing LET throughout the SOBP beam, but also with the particular cells or tissues irradiated and the dose administered. In fact, the impacts of these variables are interdependent as seen in Figures 2.5 and 2.6.16 Chinese hamster cells and xrs5, a DNA DSB repair-deficient mutant of CHO cells were exposed to x-rays or 4.2- to 266.4-MeV carbon ions, producing ionization densities of 482.7 to 13.7 keV per µm LETs. The resulting surviving fraction data were fit to the linear quadratic equation Sf = exp(-αD – βD2). In normal CHO cells, RBE increases with LET reaching a broad maximum at 175 to 275 keV per µm. For 200 keV per µm carbon ions, the RBE evaluated at the 50% cell survival level is substantially higher than that at the 1% survival level. For DSB repair-deficient xrs5 cells that exhibit a linear dose response to x-rays, there is little change in RBE with increasing LET, and the RBE is independent of the survival level at which it is evaluated. These and similar studies with heavy charged particles show that the RBE of high-LET radiation is most pronounced in the low-dose region of cells that are relatively refractory to low doses of x-rays. Linear-quadratic curve fits to dose-response data of cells that are refractory to low doses of x-rays are characterized by low α/β ratios. The effect of high-LET radiation is to linearize the survival curves by increasing α with little or no effect on β. For cells that exhibit a linear response to x-irradiation and therefore a high α/β ratio or β value of 0, as seen in Figures 2.5B and 2.6B, RBEs are relatively invariant with dose.
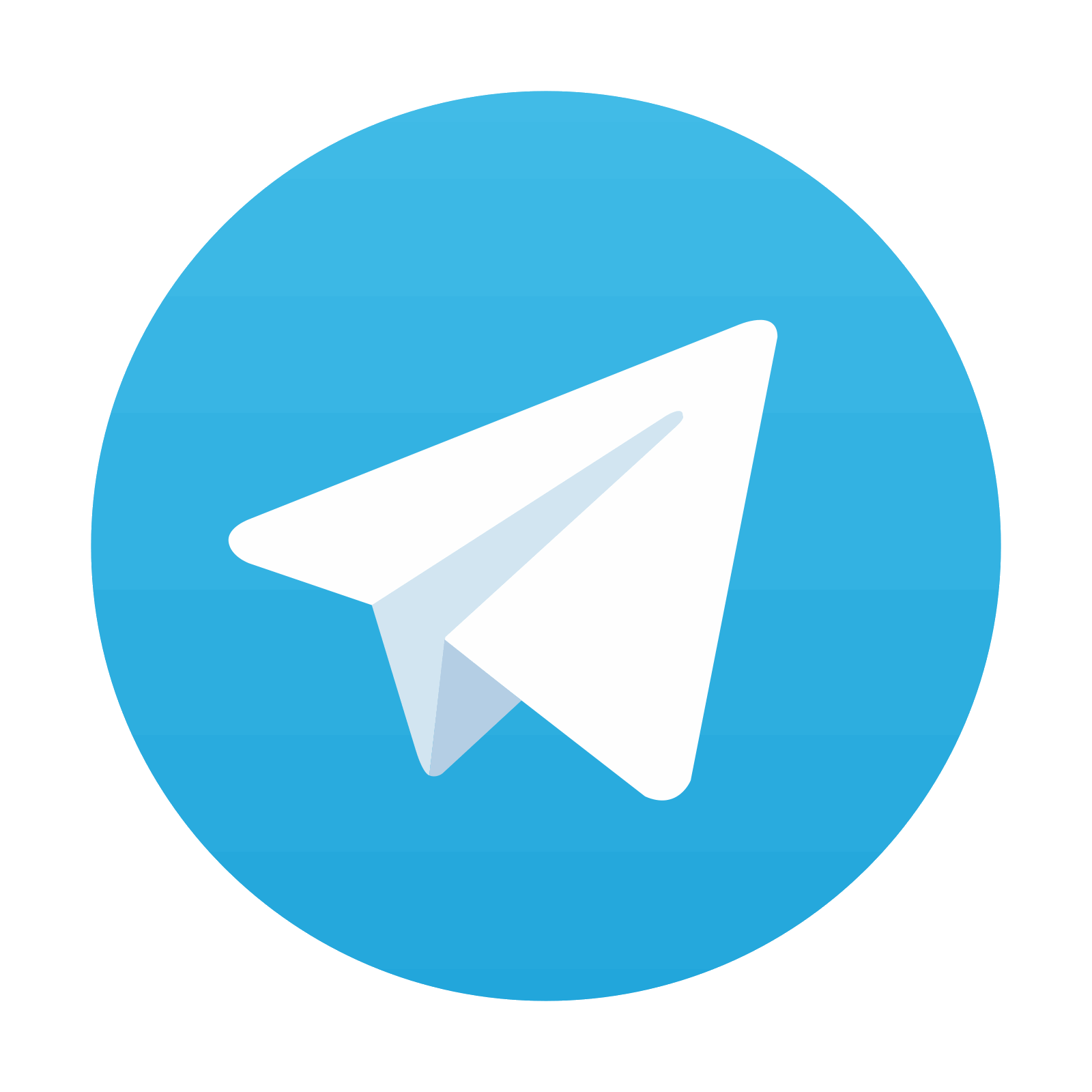
Stay updated, free articles. Join our Telegram channel
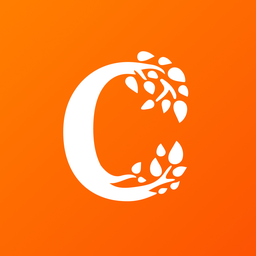
Full access? Get Clinical Tree
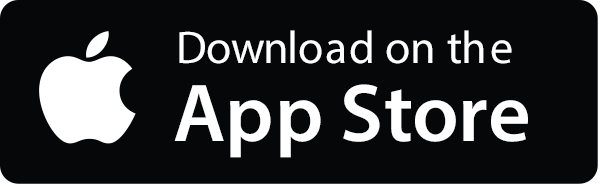
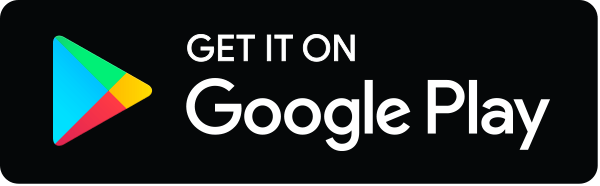