particle velocity v and acoustic pressure P is:

(21.1)
The intensity is proportional to the square of the acoustic pressure:

where |p r |2 is the absolute acoustic peak rarefractional pressure of the wave. The mechanical index MI is defined as:

where f 0 is the frequency of the acoustic wave. The spatial intensity distribution of a plane circular transducer is shown in Fig. 21.1. For LIPUS studies, the spatially and temporally averaged intensity (ISATA) is commonly reported. The latter is determined by means of the acoustic radiation force F rad acting on a large absorber that is placed perpendicular to the beam axis in the sound field (i.e., a radiation force balance):
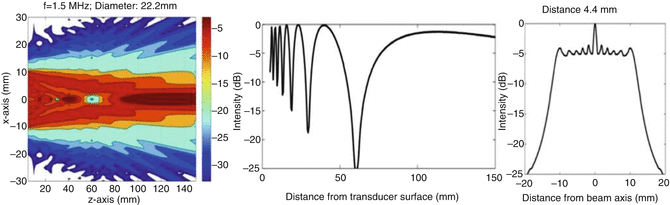

where W and c 0 are the total acoustic output power and the sound velocity in the medium, respectively. The force can be measured with an ultrasonic power balance. For example, the acoustic output power of 1 mW produces a force of 0.69 μN in water (Humphrey 2007). Total acoustic output power is related to the transducer surface area a, I SATA by:


(21.2)

(21.3)
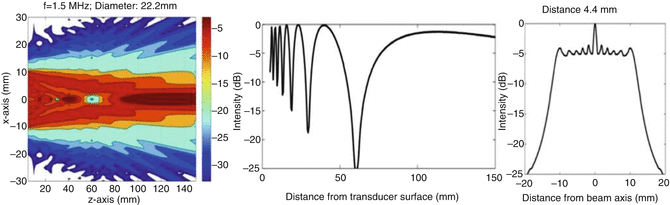
Fig. 21.1
Left: Temporal peak intensity (ITP) distribution of a plane circular transducer of 22 mm in diameter. The transducer is centered at the origin of the coordinate system in the x, y plane. Middle: Axial intensity (ITP) distribution along the beam axis (x, y = 0). Right: Lateral intensity (ITP) distribution at a distance of 4.4 mm from the transducer surface

(21.4)

(21.5)
21.2.2 Rationale for LIPUS
It is important to consider the spatial and temporal characteristics of the acoustic waveform used in LIPUS studies. For a continuous wave, the radiation force is time invariant. However, for pulsed excitation, the force varies periodically at the pulse repetition frequency (i.e., the inverse of the period between the emissions of two consecutive pulses). For the commonly used burst excitation at 1 kHz pulse repetition frequency (τon = 200 μs), the relation between temporal peak (ITP) and temporal averaged (ITA) intensity is:


(21.6)
The spatial and temporal variations of I TA within the acoustic beam give rise to local radiation pressure variations, and resulting strain and motion at frequencies independent of the emission acoustic frequency, but related to the PRF. The local radiation pressure averaged over the pulse duration τon is:
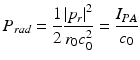
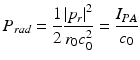
(21.7)
I PA denotes intensity averaged over the pulse duration τ on . Amplitude and direction of the radiation force depend on the properties of the interrogated material. For small-unbound particles, local radiation pressure gradients can result in particle movement. In the special case of standing waves, this can in turn lead to a separation of particles or cells at nodes/antinodes depending on the properties of the particles and fluid. This effect is utilized for example in acoustic tweezers (Ding et al. 2012). The assumption that the low frequency of the radiation force, but not the pressure alteration at the ultrasound excitation frequency, is responsible for the biological effect observed in LIPUS led to studies investigating direct stimulation at the modulating frequency of 1 kHz. This suggested that the radiation force is indeed an important component of the stimulation (Argadine et al. 2005, 2006). This may also imply that the PRF, i.e., the frequency of the resulting radiation pressure, could have an impact on the biological outcome if cells are sensitive in this range of cyclic loading, an assumption that is supported by recent experimental evidence obtained with varying PRF (Marvel et al. 2010).
Attenuation, nonlinear sound propagation (i.e., the conversion of acoustic energy from fundamental to harmonic frequencies) and divergence of the acoustic waves in the far-field of the transducer lead to a gradual decrease of the radiation pressure with increasing distance from the acoustic source. The attenuation in pure water is (Pinkerton 1949):
, whereas in soft tissues it is typically assumed that attenuation is linear with frequency and varies as ∝ = 0.3 dB/cm/MHz.

This radiation pressure gradient gives rise to a net force directed away from the transducer and a resulting fluid flow in liquids (Fig. 21.2). Solid interfaces hinder this fluid flow induced mass transport. However, it has been shown that fluid streaming can build up again in the acoustic beam behind a membrane (Humphrey 2007).
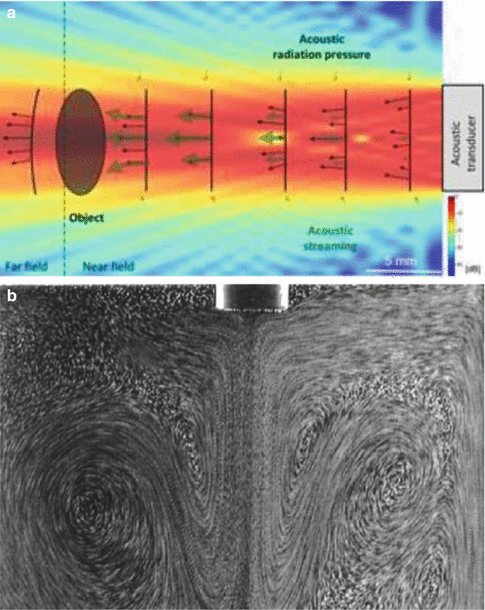
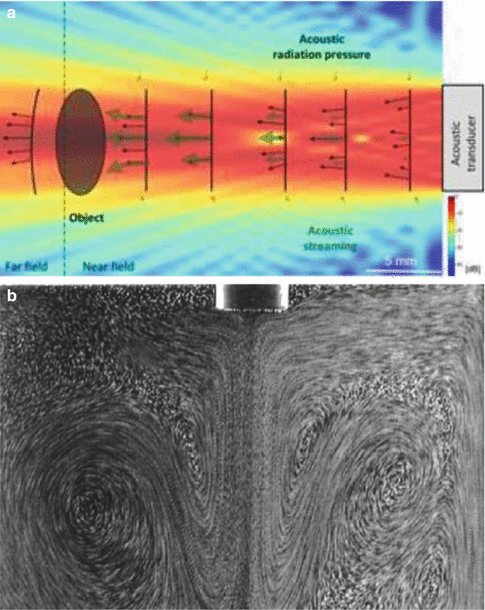
Fig. 21.2
Acoustic radiation force and streaming action on objects in the sound field generated by an acoustic source. Top: In liquids, the radiation force (black arrows) gives rise to acoustic streaming (green arrows). The inhomogeneous intensity distribution in the near field results in a regional dependence of the direction and the amplitude of the radiation force. Acoustic streaming velocity is gradually increasing from the transducer surface to the focus region. Solid interfaces interrupt the flow, but not the radiation pressure. Bottom: Acoustic streaming, visualized by ten superimposed images taken at 200 ms intervals of corn starch particles in the field of a 32-MHz plane transducer (Nowicki et al. 1998)
Experimentally, it was observed that acoustic streaming (i) increases with increasing proportion of nonlinear acoustic propagation, i.e., with the generation of harmonics at higher intensities, (ii) gradually increases with increasing distance from the transducer, and (iii) builds up 60 % of the free streaming velocity within 1 mm after solid interfaces (Humphrey 2007). The streaming velocity in water and amniotic fluid was measured at 3.5 MHz (a = 2.82 cm2, focus distance: 95 mm, CW, W = 140 mW, PA = 0.23 MPa, ISATA ~ 50 mW/cm2) to be in the order of 3 cm·s−1 (Zauhar et al. 2006). Much higher velocities (up to 9 cm·s−1) were observed for high-amplitude short pulse excitation (W = 140 mW, PA = 4 MPa, ISATA ~ 50 mW/cm2). In cell culture flasks, values of 0.4, 6.0 and 19.4 cm/s at 130, 480 and 1770 mW/cm2, respectively, were measured at 3 MHz and continuous wave excitation (Harle et al. 2005). In this later study, no cavitation events could be recorded for intensities below 500 mW/cm2.
Several groups investigated the temperature rise possibly induced by LIPUS. They generally ruled out temperature effects as the measured temperature increase was typically below 0.2 °C for intensities (SATP: spatial average, temporal peak) below 500 mW/cm2 (Harle et al. 2005) or even 2400 mW/cm2 (Li et al. 2002). This was for experimental setups where the cell dish was positioned in the focal plane with a special absorbing chamber, preventing multiple reflections within the dish (see next section). The issue of temperature rise is however highly dependent on the experimental set-up. These effects have been studied in detail by Leskinen and Hynynen (2012), reporting maximum temperature rises of 3 °C at 30 mW/cm2 (ISATA) in experimental set-ups in which the transducer was coupled to the bottom of culture plates with coupling gel. In contrast, a maximum elevation of 0.2 °C was observed if the transducer was immersed in water. This effect is related to the heating of the ultrasound transducer. The poor heat transfer properties of surrounding air leads to a heat transfer through the coupling gel into the well plate. Therefore, temperature-related biological effects may be induced in cell culture systems in which the transducer is not properly cooled, but not in systems in which the transducer is immersed in a temperature controlled water tank.
Besides temperature elevation, radiation pressures, streaming and standing waves effects, propagation of surface shear waves and Lamb waves have been reported to take place in-vitro due to mode conversions at the bottom of plastic wells (Leskinen and Hynynen 2012). These waves can in turn be responsible for bioeffects and can propagate to neighboring wells, jeopardizing the attempt to precisely control the stimulus delivered to a given cell monolayer.
It appears therefore that only cavitation can be ruled out for stimulation intensities below 500 mW/cm2. Mechanical forces resulting from spatial and temporal gradients of radiation pressure and acoustic streaming, propagation of surface and guided waves are likely sources for biological effects observed in LIPUS stimulation studies. Temperature effects can be ruled out in experimental set-ups with water coupling, but its potential role in setups using gel coupling cannot be ignored.
21.2.3 The Influence of Geometrical Configurations on In-vitro Stimulation
For the exposure the ultrasound transducer has to be coupled to the cell culture dish. Different set-ups have been used, i.e., (i) immersion of the sterilized transducer from the top directly into the culture medium in close distance (3–5 mm) to the cell layer (Fig. 21.3a), (ii) direct coupling to the bottom of the culture dish via coupling gel (Fig. 21.3b), (iii) exposure from the bottom of the culture dish with the cell layer place in the focal plane and immersion of a special sound absorption chamber (Fig. 21.3c), and (iv) the propagation of the sound through a culture flask (Fig. 21.3d).
Considering the spatial and temporal sound field dimensions, as illustrated by Fig. 21.1, and the limited dimension of the culture dishes, several sound propagation phenomena, e.g., inhomogeneous pressure distribution in the near field, multiple reflections at interfaces, standing waves and acoustic streaming have to be considered.
The sound propagation distance in the cell culture dish or flask (a few mm) is generally much smaller compared to the total propagation distance a wave can travel before it is attenuated. The attenuation in water at 1 MHz and 37 °C is very low (0.0014 dB/cm (Hensel et al. 2011)). If the incoming waves are not properly absorbed or directed away from the culture dish, they will be reflected several times at internal interfaces. Due to the long pulse duration of at least 200 μs (which is equal to 300 cycles, or in terms of wavelength: 300 × 1 mm = 0.3 m at 1.5 MHz), any interface perpendicular to the sound propagation direction, e.g., liquid/plastic, liquid/air, or liquid/transducer, will result in the development of standing waves, ring interferences and a remarkable prolongation of the duty cycle (Fig. 21.4). As a consequence, the effective peak and time average intensity levels, and the resulting modulation of the radiation force between ON and OFF phases, is considerably different from that measured in an interface-free medium. The estimation of the effects of standing waves is not straightforward as the development of nodes depends on multiple factors (Hensel et al. 2011).
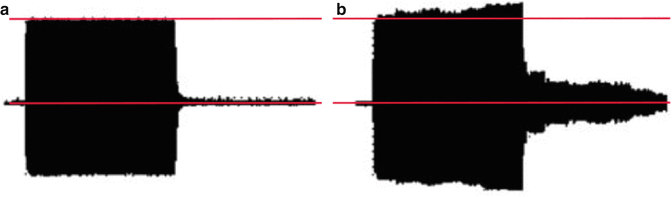
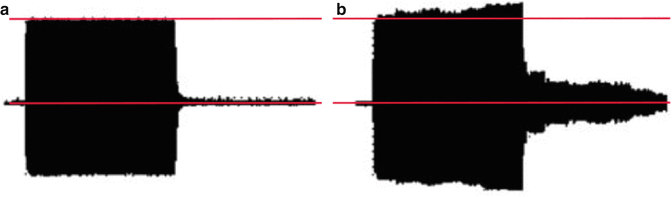
Fig. 21.4
LIPUS temporal wave pattern in a culture dish with the transducer placed on the bottom of the dish and (a) in presence or (b) absence of a silicon absorbing chamber on the top of the dish (Reproduced from Fig. 1 of Iwabuchi et al. (2005)). Without an absorbing chamber, a gradual increase of intensity during the ON cycle and a gradual decay during the OFF cycle can be seen, leading to effective slight and remarkable increases of the applied temporal peak (ITP) and temporal average (ITA) intensities, respectively
Another important factor is the transmission and reflection of the sound waves through the well plate walls. Multiple reflections inside the plastic result in pronounced oscillations of the transmitted and reflected amplitudes. The resonance frequencies for maximum transmission (f Tn ) and reflection (f Rn ) are:
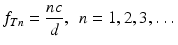
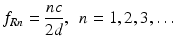
where c and d are sound velocity and thickness of the plate, respectively.
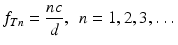
(21.8)
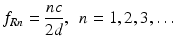
(21.9)
If the ultrasound frequency is set to the anti-resonance value, the transmitted or reflected wave amplitudes can be completely diminished (Leskinen and Hynynen 2012). For a typical polystyrene well-chamber with a longitudinal sound velocity of 2305 m/s at 37 °C and a well bottom thickness of 1.22 mm, the transmission and reflection resonance frequencies are at intervals of 1916 and 958 kHz, respectively. Therefore, stimulations conducted with the same set-up, but different frequencies, may result in different exposure levels in the well, if the sound has to be propagated through the well plate bottom.
21.2.4 The LIPUS ‘Dose’ and the Need for Standardization
Standardization of experimental settings, both in-vitro and in-vivo, is a crucial factor in order to not only compare studies, but also to be able to identify relevant parameters and subsequent physical and biological effects. This is particularly important in-vitro because both the positioning of the culture plates/wells with respect to the ultrasound source and the output of the source can be regarded as responsible for a lack of reproducibility of LIPUS treatments of cells. In particular, the formation of standing wave patterns have been reported to be very sensitive to the height of the fluid in the wells, and to be responsible for very large spatial variations of the peak pressure applied on the cells (Williams et al. 2013; Hensel et al. 2011). The effects of intensities have indeed been reported to influence the outcome of in-vitro experiments.
The concept of an acoustic ‘dose’ for LIPUS that could be standardized is difficult to derive. Similarly to therapeutic thermal ultrasound (Duck 2011), the dose should be related to the amount of bioeffect induced. However, given the lack of understanding of which parameters of the stimulation are responsible for any given bioeffects, the dose is so far a lacking concept in the field of LIPUS. We therefore suggest that precise data on transducer geometry, intensity, peak pressure, duty cycle, pulse duration, center frequency are reported. Concerning the in-vitro cases, great care should be given to the geometry of the experimental set-up to evaluate the aforementioned parameters in the well and in particular to avoid the formation of standing wave patterns and heating by the transducer. For historical reasons, most of the studies have been performed using parameters similar to the Exogen commercial system, i.e.,: plane transducer, ISATA = 30 m W/cm2, 200 μs pulses, 1 kHz PRF, treatment time 20 min daily. Unless otherwise mentioned, our discussion is focused on data obtained using these settings.
21.3 Potential Bio-effects of LIPUS
The difficulty in interpreting LIPUS experiments lies in the complexity of the triggered physical phenomena that can in turn potentially induce bio-effects. Compared to experimental designs implying laser traps, controlled fluid shear stress or magnetic bead twisting, it is difficult to isolate a single pattern of mechanical stimulation by a propagating ultrasound wave. This problem is even more severe as several of these potential acoustical phenomena can be activated with similar acoustic output parameters to a variable extent, depending on the particular geometry of the stimulation system, (Hensel et al. 2011) and that the biological response to the treatment may depend on cell and tissue types (Claes and Willie 2007).
Potential LIPUS physical effects that may induce a biological response can be divided into thermal and non-thermal effects.
21.3.1 Thermal Effects
Temperature rises associated with LIPUS can range from a few tenths of a degree, to a few degrees at the bottom of the culture well when coupling gel is used (Leskinen and Hynynen 2012). These changes can be significant enough to regulate thermo-sensitive enzymes, such as metalloproteinase, which support threefold reaction rate increases per 2 °C temperature rise and are important enzymes for bone matrix remodeling (Kusano et al. 1998; Welgus et al. 1981). These associated effects should be further investigated. For water-coupling experimental setups, it is a fair assumption to consider that thermal effects are not determining, but rather additive to the non-thermal effects.
21.3.2 Non-thermal effects
Non-thermal effects imply dynamic mechanical forces at various frequencies (from quasi-stationary up to the acoustic excitation frequency) (Sarvazyan et al. 2010; Argadine et al. 2005, 2006). A bulk ultrasonic wave is a propagating mechanical perturbation of the medium, and for a frequency of 1 MHz (typical center frequency for LIPUS devices) the corresponding wavelength is about 1.5 mm in soft tissues or in culture medium. This wave can directly affect the mechanosensitive elements (see next section) by inducing oscillatory strains at very high frequency (typically thousands to a few million oscillations per second) compared to physiological strains. The strain amplitudes will be a function of the applied intensity, and are typically of the order of 10−5 for low intensity ultrasound. Secondary mechanisms at lower frequencies can also be triggered by the acoustic radiation force (Sarvazyan et al. 2010) – an oscillatory strain acting at the frequency corresponding to the pulse repetition frequency for pulsed ultrasound (typically 1 kHz for LIPUS-type stimulation), resulting in a low frequency cyclic mechanical stimulus (Argadine et al. 2005, 2006). Inhomogeneities in the acoustic near field, or caused standing waves and ring interferences, can also generate local strain gradients. Finally, fluid-flow related phenomena can also be induced, such as acoustic streaming, resulting in a quasi-stationary fluid flow (Zauhar et al. 2006) and microstreaming, i.e., fluid streaming around oscillating particles.
The relevant scale at which these effects will influence the cellular and tissue biological responses in the context of bone healing remains an open question.
21.3.3 Effects at the Tissue and Cellular Scales
Radiation force, fluid flow and strain gradients can create shear stresses on cell membranes. Acoustic streaming and microstreaming, often used in the literature interchangeably but consequences of two separate physical phenomena, are thought to play important roles in LIPUS action, especially in-vitro. Acoustic streaming. The former is associated with a transfer of momentum to the fluid that will result in nutrient redistribution in the culture medium in-vitro and perturbation of local homeostasis, thus triggering biological responses. Microstreaming The latter is generated in response to oscillating gas bubbles, or other small acoustic inhomogeneities, causing circulatory fluid movement. Microstreaming around gas bubbles in response to LIPUS is not likely to occur in bone tissue or in the fracture callus in-vivo because the pressure levels applied by LIPUS systems are well below cavitation thresholds. However, it can be hypothesized that the wave propagation through the highly porous network of soft and mineralized tissues in the fracture callus may induce fluid micromovement in the pores. Fluid flow, created by either acoustic streaming or microstreaming, can modulate the extracellular matrix and can apply shear stress, in turn activating mechanoreceptors on the cell membranes.
21.3.4 Intracellular Effects
Direct mechanical action of the ultrasound wave on cell membranes or proteins may trigger a biological response. At intensities between 1 and 2 W/cm2 (i.e., much higher than applied by typical LIPUS), the very low strains induced by ultrasound on cells in-vitro have been reported to induce a prompt fluidization of the cytoskeleton, resulting in accelerated cytoskeletal remodeling events (Mizrahi et al. 2012). These mechanisms, similar to those caused by physiological strains of higher amplitude and much lower frequency, could contribute to LIPUS bioeffects.
Other proposed mechanisms of pulsed ultrasound action at the intracellular scale include the hypothesis of relative oscillatory displacements between intracellular elements of different densities (Or and Kimmel 2009), such as cell nuclei and the structures in which they are embedded. By modeling the linear oscillations of such structures with different rheological models, Or and Kimmel (2009) suggested that LIPUS can trigger cyclic intracellular displacements. These are comparable with, and even larger than, the mean thermal fluctuations, with resonance frequencies in the range of tens to hundreds of kHz, so within the range of direct oscillatory movement caused by the ultrasound wave or by the acoustic radiation pressure. Additionally, the ‘bilayer sonophore’ model (Krasovitski et al. 2011) they proposed describes a mechanism where the intramembranous hydrophobic space between the two lipid monolayer leaflets inflates and deflates periodically when exposed to ultrasound. Accordingly, being pulled apart during the depression phase (negative pressure) of an ultrasound cycle, and pushed back together by the positive pressure. Here, the main assumption being that negative acoustic pressures are large enough to overcome the molecular attractive forces of the two cell membrane leaflets. Published data showed that this effect can occur at levels below cavitation thresholds, but were obtained with intensity levels higher than typically used in LIPUS treatment (1 W/cm2 at 1 MHz). Nevertheless, this is an interesting model that would merit further investigation with LIPUS-type ultrasound fields.
Another mathematical model was proposed to predict ultrasound-induced intra-cellular stresses and strain using a biphasic (solid elastic structures and macromolecules on one side, fluid cytosol on the other side) description of the cells in a harmonic standing wave field (Louw et al. 2013). Modeling results predict that stresses and strain are maximized within the cell at two distinct resonant frequencies, which are cell-type dependent through the geometric and mechanical characteristics of its different components. Stress gradients are induced through dilatational deformation, resulting in net forces acting on the nucleus, possibly triggering transduction by the nucleus and leading to load-inducible gene expression. Stimulated load-inducible gene expression should therefore be maximized when excitation frequency matches the cell resonant frequency, a prediction that was confirmed by experimental data (Louw et al. 2013).
21.3.5 Molecular Effects
At the molecular scale, it is well known that ultrasound can interact with molecules. This interaction is used in the field of “molecular acoustics” to probe molecular properties, based on the measurement of variations in ultrasound velocity (Sarvazyan 1991) or absorption (Morse et al. 1999). These measurements utilize the changes in molecular compressibility (and in ultrasound velocity) or in ultrasound absorption following changes in conformation, in solvent-molecule interactions or in hydration. All these effects are the results of intra and intermolecular forces, interaction potentials, electrostatic interactions, rigidities of interatomic bonds and relaxation phenomena. For example, lipids, constituting the second most prevalent component of dry weight of many tissues after proteins, interact with ultrasound. Lipids and structures associated with the cell membrane exhibit an absorption behavior for ultrasound, probably linked to relaxation phenomena with relaxation frequencies in the range 1–16 MHz. In large unilamellar vehicles, these relaxation phenomena are linked to the interaction of ultrasound with the hydrophobic side chains, leading to a structural reorganization of small molecular domains (Morse et al. 1999). These interactions can affect the function of biological molecules. At very high intensities, ultrasound can damage biological molecules, e.g., DNA degradation in the presence (Elsner and Lindblad 1989) or in the absence of cavitation (Hawley et al. 1963). Nonetheless, these intensities are much higher than those typically used in low intensity pulsed ultrasound stimulation or other techniques mentioned in this review (for an in-vivo use of ultrasound, safety guidelines are provided by scientific societies or regulatory agencies in order to avoid such effects). At lower intensities however, where absorption is a result of ultrasound interaction with molecules, can the deposited ultrasound energy induce transient conformational changes, or changes in molecule-molecule or molecule-solvent interactions, which in turn could modify the biological functions of these proteins? It has been suggested that ultrasound could disrupt multimolecular complexes (Johns 2002), although intensities and mechanisms (cavitation or not) at which these phenomena could occur were not specified. A change of protein conformation is believed to be responsible for cytoskeleton fluidization observed after low intensity therapeutic ultrasound treatment at 1 MHz center frequency, with 20 % duty cycle and pressures in the order of 200 kPa (Mizrahi et al. 2012). The origin of these phenomena is thought to be due to the local strains applied by ultrasound that could be large enough to disrupt weak nonspecific bonds, altering protein conformation and triggering structural remodeling of the cytoskeleton.
21.3.6 Other Effects
Finally, some reported that ultrasound bioeffects are difficult to categorize due to the lack of knowledge of the physics underlying them. In particular, ultrasound can affect intracellular trafficking, and this would be relevant in the stimulation of bone healing processes. It has been recently reported that after a few minutes of exposure to 1 MHz ultrasound (at ISATA of 0.3 W/cm2, 50 % duty cycle and 5 Hz PRF), cells that had already internalized plasmids (delivered using calcium phosphate co-precipitates) expressed higher transfection rates than the non-ultrasound treated cells (Hassan et al. 2012). The mechanisms of these effects are not clearly understood. Plausible explanations are either a destabilization of the endosomal vesicles or an increase in the net diffusivity of the pDNA through the cytoplasm, an inhibitory action on enzymatic levels or assistance in the permeabilization of the nuclear envelope. Whatever the exact mechanism, this study clearly demonstrated that ultrasound levels below cavitation thresholds can modulate intracellular trafficking of pDNA, and by extension could more generally modulate intracellular trafficking of other molecules, thereby eventually triggering or enhancing a possible mechanoresponse.
21.4 LIPUS and Mechanotransduction
Conventionally, ‘mechanotransduction’ is the process in which specific cellular machineries switch a physical stimulus into chemical activities to trigger downstream signaling. Conformational changes in proteins, such as stretch-activated ion channels or mechanosensitive adhesion structures, often mediate conversion of force into chemical signaling. Given the modalities of mechanical stimulation of LIPUS shown in Sect. 21.4, it is difficult at this stage to specify a biological response to a particular strain range. Nevertheless, the magnitude of interfragmentary movement, the rate and timing of micromotion application and the number of loading cycles are mechanical boundaries susceptible to impact signal transduction pathways, and subsequently gene up- or down-regulation, thereby influencing cell phenotype and function.
A variety of mechanosensitive membrane molecules and microdomains have been identified, including ion channels, receptors, G-proteins, adhesion molecules, caveolae, the glycocalyx and primary cilia. As the intracellular cytoskeleton ultimately bears the impact of force applied on cells, it also represents a major class of mechanosensitive structures. The activation, reinforcement and/or redistribution of membrane-bound complexes, and the reorganization of the cytoskeleton and extracellular matrix, are all immediate responses to forces. These events critically influence cell metabolism, protein synthesis, cell survival and stem cell commitment, in addition to migration, spreading and contraction. Compared to other types of mechanical stimuli (such as shear stress or extracellular deformation), ultrasound-induced mechanotransduction pathways are less well defined. We will present mechanosensitive structures that should potentially come into play knowing that the sensor molecules that initially sense the different varieties and orders of magnitude of mechanical stimuli are not fully defined.
Changes in free intracellular calcium concentration are one of the first responses against environmental stress and are important biological signals in mechanotransduction: Upon ion channel activation, calcium is mobilized into the cytoplasm from outside the cell membrane or from intracellular reservoirs, including endoplasmic reticulum, sarcoplasmic reticulum and mitochondria. There is experimental evidence suggesting that ultrasound, even at low intensity, increases intracellular calcium concentration (Parvizi et al. 2002), and that blocking this rise with intracellular calcium chelating agents or inhibiting Ca2+/ATP-ase, abolishes the stimulatory effect of ultrasound on proteoglycan synthesis by chondrocytes. Proliferative LIPUS effects were also shown to depend on the release of ATP/purines through Ca2+ and P2Y receptors (Alvarenga et al. 2010). Calcium is a product generated indirectly from enzymatic activities, such as phospholipase C. In consequence of the intracellular calcium increase, calcium acts as a secondary messenger by allosterically binding to proteins including calmodulin, troponin-C and annexin, as well as calpain, and then the complex triggers downstream cellular processes. Recently, it has been shown that Ca2+ signals are oscillatory (Kim et al. 2009), and that these oscillatory Ca2+ signals are crucial for a variety of cellular functions, such as bone marrow-derived mesenchymal stem cell (HMSCs) differentiation (D’Souza et al. 2001; den Dekker et al. 2001). In HMSCs, calcium oscillation can be regulated by electrical stimulation (Sun et al. 2007) or substrate rigidity (Kim et al. 2009). In this later study, Ca2+ oscillation occurred via the RhoA GTPase pathways. These are major cytoskeleton regulators, although sometimes not correlated with cytoskeleton activities (Kobayashi and Sokabe 2010). However, at this stage we do not have evidence that oscillatory Ca2+ signals are a target for LIPUS stimulation, and further studies should address whether intracellular calcium rise is a primary target or a downstream event.
Another major factor in mechanotransduction of adherent cells, being at the center of cell integration of internal and external signals, is the “cytoskeleton-focal adhesion-extracellular matrix (ECM) connection”. Integrin-mediated focal adhesions (FAs) are large, multi-protein complexes that link the actin cytoskeleton to the ECM and take part in adhesion-mediated signaling. FAs insure cell adhesion to ECM via integrin-regulated organization. They undergo maturation wherein they grow and differentially change composition to provide traction and to transduce the signals that drive cell migration. This is crucial to various biological processes, including wound healing. FAs are related to signaling networks and dynamically modulate the strength of the linkage between integrin and actin, and control the organization of the actin cytoskeleton (Kuo et al. 2011; Schiller et al. 2011). In response to mechanical force, these force-sensitive focal adhesion proteins may undergo structural rearrangement or enzymatic modifications that change their binding preferences with respect to other associated proteins. This then further modulates the protein association with focal complexes (del Rio et al. 2009). It has been shown that forces in the physiological range (2–20 pN) are sufficient to stretch FA molecules (such as talin), exposing cryptic binding sites for others molecules (such as vinculin), suggesting a role for protein binding in the talin-vinculin system in the mechanotransduction process. LIPUS-type mechanical stimulation has been shown to alter the role of integrins (Whitney et al. 2012). It was shown to activate the integrin/phosphatidylinositol 3-OH kinase/Akt pathway which is associated with cell proliferation (Takeuchi et al. 2008). It was also shown to trigger phosphorylation of FA signaling molecules, such as FAK, Src and p130Cas, which in turn phosphorylate and activate Erk and MAP Kinases. LIPUS signal transduction to the nucleus via the integrin/MAPK pathway influences numerous cellular processes, including migration and a wide range of gene regulations (Whitney et al. 2012; Sato et al. 2014).
Integrin-mediated signals can be modulated by changes in ECM characteristics (composition/mechanical properties). ECM compounds were also stimulated by LIPUS exposure: Type IX-collagen in chondrocytes in 3D matrices (Takeuchi et al. 2008), proteoglycans and growth factors with their receptors (BMP2, FGF7, TGF-β R, EGFR, VEGF) in nucleus pulposus cell line (Kobayashi et al. 2009). However, other potential candidates should be investigated, including (i) stretch-activated ion channels or G-protein coupled receptors (Liedert et al. 2006), (ii) the glycocalyx, a pericellular GAG-proteoglycan rich layer surrounding the cell membrane that creates a drag force when fluid flow passes over causing plasma membrane deformation (Morris et al. 2010), and (iii) the primary cilium, an immotile microtubule-based organelle that protrudes like an antenna from the apical cell surface. This already has implications as a mechanosensor in a variety of cell types, including mesenchymal stem cells MSCs (Malone et al. 2007).
Along with transmembrane integrins, direct cell-to-cell communication is important for mechanotransduction. The connexins not only experience the different biomechanical forces within the system, but also act as effector proteins in coordinating responses within groups of cells against these forces. Improved cell-to-cell communication via gap junctions assessed by dye transfer assay was reported after LIPUS exposure in rat MSCs. Inhibition of gap junctions led to decreased activation of Erk1/2 and p38 MAPK kinases, and attenuated alkaline phosphatase (ALP) activity in response to LIPUS, implying that gap junctions are essential for LIPUS effects on osteogenic differentiation of MSCs (Sena et al. 2011). Activation of p38 and Erk1/2 MAPK kinases in rat MSCs, and Erk1/2 MAPK kinase in murine pre-osteoblastic MC3T3-E1 cells, has been confirmed by other investigators (Angle et al. 2011; Bandow et al. 2007).
Activation of another mechanoreceptor on the surface of osteoblasts, angiotensin II type I receptor (ATI), has been suggested by Bandow et al. (2007). ATI receptor expression increased with osteoblast maturation, and inhibition of the receptor resulted in ablation of LIPUS-induced cytokine expression and Erk1/2 phosphorylation. To date, integrins remain the most studied receptors known to transmit ultrasound-induced signals into cells. Further investigation is required into other mechanoreceptors of which their mechanofunction is not well understood, like ATI, as well as more detailed function elucidation of signaling pathways transmitting the converted intracellular mechanical stimuli.
Among genes whose expression is promoted by LIPUS are chemokines such as monocyte chemoattractant protein (MCP)-1, macrophage-inflammatory protein (MIP)-1 and RANKL in osteoblastic cells (Bandow et al. 2007), and TNF-α in macrophagic cells (Iwabuchi et al. 2008). In line with these results, LIPUS inhibits osteoblast lipopolysaccharide-induced inflammatory responses through TLR4-MyD88 dissociation (Nakao et al. 2014).
Mechanical loading plays a key role in bone formation and maintenance. While unloading induces osteocyte apoptosis and bone loss in-vivo, mechanical stimuli prevent osteocyte death through a mechanism involving beta-catenin accumulation and ERK nuclear translocation, establishing osteocytes as primary mechanosensors (Temiyasathit and Jacobs 2010). To understand the cellular mechanisms underlying LIPUS on enhanced fracture healing in a rat model, Fung et al. (2014) investigated the effect of ultrasound axial distances on osteocytes and mechanotransduction between osteocytes and pre-osteoblasts (bone-forming cells) through paracrine signaling. They demonstrated the positive effects of far field LIPUS on stimulating osteocyte nitric oxide production and promoting mechanotransduction between osteocytes and osteoblasts.
Although much work has focused on dissecting the adhesive and structural components of the cell responsible for transducing external mechanical forces into biochemical signaling cascades, how mechanical signals are transmitted to the nucleus and activate specific gene expression programs have only been recently suggested. One necessary step in these processes is the transport of signaling molecules from the cytoplasm to the nucleus; a common theme in mechanotransduction (Sharili and Connelly 2014). The SRF (serum-response factor) and YAP (Yes-associated protein)/TAZ (transcriptional co-activator with PDZ-binding motif) pathways are known mediators of this process in multiple cell types, including mesenchymal stem cells (Costa et al. 2012). In addition, recent evidence suggests a potential role of YAP/TAZ for β-catenin and Smad signaling in mechanotransduction, both critical for osteogenic differentiation (Halder et al. 2012). YAP/TAZ accumulates in the nucleus of cells cultured on stiff gels or large ECM micropatterns, and promotes osteogenic MSC differentiation (Dupont et al. 2011). This response requires RhoA and cytoskeletal contractility, preventing YAP/TAZ from being targeted for degradation by phosphorylation. It has been established that LIPUS influences the multilineage differentiation of mesenchymal stem and progenitor cell lines through a ROCK dependent pathway (Kusuyama et al. 2014). YAP/TAZ nuclear translocation under mechanical stimulation therefore provides another mechanism for linking biophysical cues to transcriptional responses and cell differentiation. To our knowledge, there is no evidence that LIPUS could activate YAP/TAZ in exposed cells, but it could represent a clear demonstration of mechanosensory pathway activation by LIPUS.
It is also important to consider that continuous mechanical stimulation received by cells can be deleterious for integrity of large structures, such as microfilaments or microtubules, but also for individual proteins. In muscle cells, contractile apparatus is subject to intense mechanical stress, leading to irreversible protein 3D structure modification, such as the actin-crosslinking protein filamin. A chaperone dependent process facilitates the degradation of damaged filamin through selective autophagy. Recent seminal work in Jorg Hohfeld’s lab identified a chaperone-assisted selective autophagy (Klionsky et al. 2012) as a tension-induced autophagy pathway (Arndt et al. 2010; Ulbricht et al. 2013). A mechanosensitive chaperone (BAG3) utilizes its WW domain to engage in YAP/TAZ signaling in order to stimulate filamin transcription to maintain actin anchoring and crosslinking under mechanical tension (Ulbricht and Höhfeld 2013). By integrating tension sensing, via autophagosome formation and transcription regulation during mechanotransduction, the chaperone-assisted selective autophagy (CASA) machinery ensures tissue homeostasis and regulates fundamental cellular processes, such as adhesion, migration and proliferation. In this context, it is conceivable that LIPUS exposure could alter many mechanosensitive proteins, leading to their recycling and renewal using a CASA mechanism. Activation of autophagy by LIPUS may explain many, if not all, of the above-described mechano-mimetic effects, especially on stem cell differentiation.
Furthermore, how LIPUS-induced cues from the nanoscale- to the tissue-level compromise tensional homeostasis still need to be investigated to provide a comprehensive understanding of coordinated mechanoresponsiveness.
21.5 LIPUS-Induced Bone Healing: Biological Evidence
Bone healing is a complex biological phenomenon composed of a temporally and spatially overlapping sequence of four basic stages: Inflammation, soft callus formation, bone formation and bone remodeling. To some extent these stages recapitulate the development of bone formation processes (Gerstenfeld et al. 2003, 2006). LIPUS has been reported to actively influence all these stages, i.e., (1) mitigation of the soft tissue phases (inflammation and soft callus formation), (2) potential acceleration of the onset of bone formation and (3) influence of the biomechanical properties of remodeled bone (Azuma et al. 2001). There are various ways by which LIPUS might play a role in this orchestrated sequence of events, affecting migration, proliferation, differentiation of several cell types (e.g., MSCs, osteoblasts, chondrocytes, osteoclasts, fibroblasts, endothelial cells and inflammatory cells), and ECM production and remodeling. In particular, MSCs possess multiple lineage differentiating potentials, and can form cells from bone, cartilage, fat, muscle, tendon etc. They are therefore receiving more and more attention in the field of regeneration therapies (Pittenger et al. 1999).
Numerous phenomenological studies have investigated cellular events in response to LIPUS exposure. Figure 21.5 illustrates possible ultrasound effects on the sequence of events in a healing bone fracture, summarizing gene expression and release of chemical messengers in response to ultrasound as determined by in-vitro studies. This is described in more detail in Sect. 21.5.1. Furthermore, signaling pathways generated by LIPUS important for transduction of mechanostimuli are discussed in Sect. 21.5.3 and summarized in Table 21.1. Lastly, the possible role of ultrasound in maintenance of extracellular fluid homeostasis is discussed in the Sect. 21.5.4.
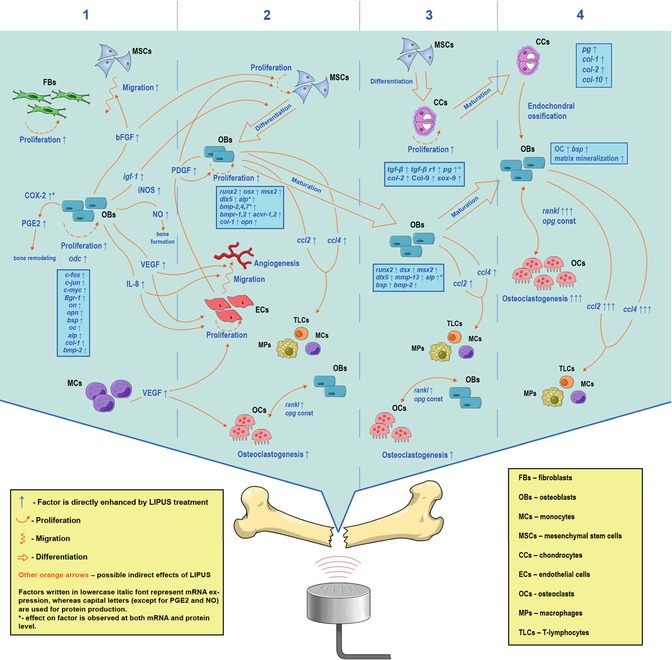
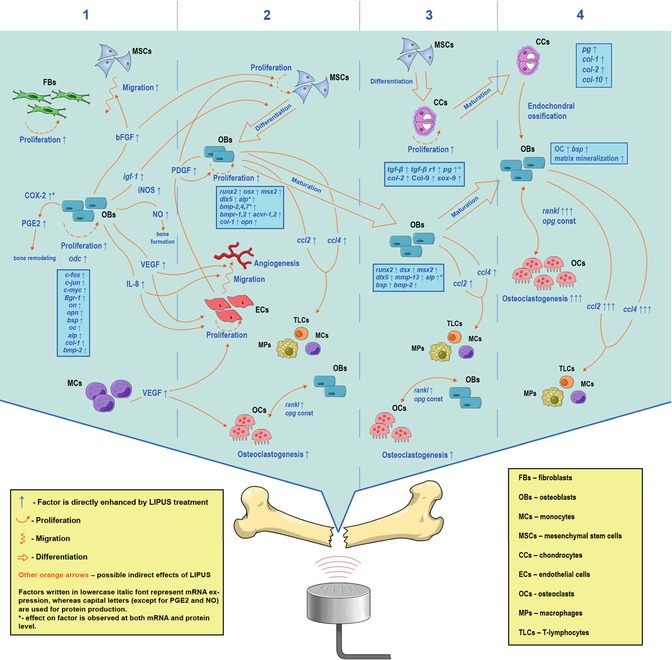
Fig. 21.5
Summary of hypothetical LIPUS effects on cellular events from published in-vitro data. The columns represent the four phases during in-vivo endochondral bone fracture healing: Phase 1 – early events soon after the bone injury, i.e., hematoma formation, inflammation and migration of osteogenic precursors; phase 2 – angiogenesis, proliferation of MSCs and osteoblasts, and osteogenic differentiation; phase 3 – chondrogenesis and maturation of osteoblasts; phase 4 – maturation of chondrocytes, woven bone formation and remodeling
Table 21.1
Ultrasound-induced intracellular signaling pathways
Reference | Signaling molecule | US signal/conditions | Cells | Exposure time | Time of the effect | Additional information |
---|---|---|---|---|---|---|
Zhou et al. (2004) | RhoA-GTP ↑ p-Erk1/2 ↑ | 1.5 MHz, 30 mW/cm2 200 μs burst, 1 kHz PRF Transducer is from the bottom via coupling gel | Human primary skin fibroblasts | 11 min | Peak after 10–20 min | These signaling molecules are suggested to be involved in US-induced DNA synthesis |
Mahoney et al. (2009) | Rac ↑ | 1.5 MHz, 30 mW/cm2 1 kHz PRF Transducer is from the bottom via coupling gel | Mouse embryonic fibroblasts (WT & syndecan-4 −/− mutant) | 30 min | 30 min post-US | PKCα, mediating Rac activity in the presence of syndecan-4, was not involved in US-induced activity of the protein |
Tang et al. (2006) | p-FAF ↑ p-85 of PI3K ↑ p-Akt ↑ Activation of canonical NF-κB pathway PGE2 ↑ | 1.5 MHz, 30 mW/cm2 200 μs burst, 1 kHz PRF Transducer is from the top, 5–6 mm away from the cells | MC3T3-E1 mouse preosteoblasts, primary osteoblasts | 20 min | Peak after 10–30 min 6 h post-US Peaking at 24 h | The activation of the listed pathways is implicated with enhanced COX-2 expression, which is supported by higher secretion of PGE2 in the medium |
Ren et al. (2013) | p-p38 ↑ | 1 MHz, 90 mW/cm2 (not clear how it is pulsed) | Human periodontal ligament cells | 20 min | Peak after 30 min and sustained for 6 h | Inhibition of p-38 reduces US-increased ALP activity, OC secretion and matrix mineralization |
Hou et al. (2009) | Ras ↑ p-Raf-1 ↑ p-MEK1/2 ↑ p-Erk1/2 ↑ p-IKKα/β ↑ p-IκBα ↑ p-p65 ↑ | 1.5 MHz, ISATA = 30 mW/cm2, 200 μs burst at 1 kHz PRF, transducer is from the top, 5 mm away from the cells | MC3T3-E1 mouse pre-osteoblasts | 20 min | 15 min post-US 30 min post-US | US increased activation of the listed signaling molecules, resulting in up-regulated iNOS production |
Ikeda et al. (2006) | p-ERK 1/2 ↑ p-p38 ↑ p-p38 ↑ | 1.5 MHz, 70 mW/cm2, 2 ms burst 100 Hz PRF Transducer is from the top, 3–4 mm away from the cells | C2C12 mouse myoblasts | 20 min | 60 min post-US Starting at 10 min Peaking at 30 min 10 min post-US | The pathways drive cell differentiation into chondroblastic/osteoblastic lineages |
Takeuchi et al. (2008) | p-Akt ↑ p-ERK 1/2 ↑ | 1.5 MHz, 30 mW/cm2, 200 μs burst, 1 kHz PRF Transducer is from the bottom via coupling gel | Primary pig chondrocytes in 3D collagen matrix | 20 min/day for 7 days | 2 h post-US on day 7 | Akt pathway is involved in US-increased chondrocyte proliferation, which is confirmed by Cyclins D1 & B1 up-regulation |
Whitney et al. (2012) | p-FAK (Y397) ↑ p-Src (Y416) ↑ p-p130Cas (Y249) ↑ p-CrkII (Y221) ↑ p-Erk1/2 ↑ | 5 MHz in continuous mode, Spatial averaged pressure 14 kPa, Transducer immersed in the media 6 mm away from the bottom | Human primary chondrocytes | 3 min | 60 min post-US 5 min post-US Decrease by 60 min | Integrins and Src inhibitors lowered US-induced Erk1/2 phosphorylation, implying that they function upstream from the protein. |
Parvizi et al. (2002) | Intracellular [Ca2+] ↑ | 1 MHz, 111–450 kPa, 200 μs burst, 1 kHz PRF | Neonatal rat primary chondrocytes | 2 s-10 min | Right after US | Transient increase at 111 kPa and sustained rise at 450 kPa. Increased [Ca2+] was established to be implicated in US-induced proteoglycan synthesis |
Sena et al. (2011) | p-Erk1/2 ↑ p-p38 ↑ | 1.5 MHz, 30 mW/cm2, 200 μs burst, 1 kHz PRF Transducer is from the bottom via coupling gel | Rat bone marrow stromal cells | 20 min | 30 min post-US | The inhibition of gap-junctions abolishes US-enhanced p-Erk1/2 and p38 phosphorylation |
Angle et al. (2011) | p-Erk1/2 ↑ p-p38 ↑ | 1.5 MHz, 2, 15 or 30 mW/cm2, 200 μs burst, 1 kHz PRF | Rat bone marrow stromal cells (in osteogenic media) | 20 min | 30 min post-US | More intense p-p38 phosphorylation is seen at 15 mW/cm2, whereas p-Erk1/2 phosphorylation is only more pronounced at 30 mW/cm2 |
Bandow et al. (2007) | p-Erk1/2 ↑ | 1.5 MHz, 30 mW/cm2, 200 μs burst, 1 kHz PRF Transducer is 13 cm away from the bottom in the water tank | Mouse MC3T3-E1 cells (3 weeks differentiated) | 20 min | 5 min post-US | AT1 receptor inhibitor down-regulates LIPUS-induced enhanced Erk1/2 activation |
21.5.1 LIPUS-Stimulated Gene Expression and Signaling Molecule Release
Figure 21.5 depicts the effect of ultrasound on each stage of fracture healing. The data have been gathered from in-vitro reports and are presented in the figure within four distinct phases corresponding to the four basic stages of the bone healing process. Phase 1 illustrates the possible early effects of LIPUS observed soon after the bone injury, including hematoma formation, inflammation and migration of precursor cells. Phase 2 shows LIPUS-effect on angiogenesis, MSC proliferation, osteoblast proliferation and differentiation. Phase 3 depicts LIPUS-enhanced chondrogenesis and osteoblast maturation. Phase 4 represents further differentiation of chondrocytes, LIPUS-accelerated woven bone formation and remodeling. The information gathered in Fig. 21.5 and in Table 21.1 does not recapitulate the entire complexity of the biological mechanisms underlying the response to ultrasound treatment, but rather represents an attempt to reconstitute a hypothetical consequence of cellular events which were observed during in-vitro studies performed in various cell types and species. We will now review this data in more detail.
21.5.1.1 Inflammation
Soon after the bone fracture, fibroblasts form a granulation tissue to support the damaged site and a hematoma is formed. The influence of ultrasound stimulation on proliferation of fibroblasts within 24 h post-exposure has been reported in several studies (Zhou et al. 2004; Doan et al. 1999; Reher et al. 1998). Hematoma formation is accompanied by the release of chemotactic factors, recruiting inflammatory cells, MSCs and other progenitors to the fracture site. Kumagai et al. (2012) reported recruitment of local osteoprogenitors and osteogneic precurors from systemic circulation to the fracture site in an in-vivo mouse model after LIPUS treatment (Fig. 21.5, ph. 1).
21.5.1.2 Angiogenesis
It has been shown that LIPUS up-regulates interleukin-8 (IL-8) secretion in human mandibular osteoblasts (Doan et al. 1999) and IL-8 gene expression in murine pre-osteoblasts (Bandow et al. 2007) (Fig. 21.5, ph. 1). IL-8 is a cytokine known to induce endothelial cells proliferation and migration, which, in turn, are crucial for new blood vessel formation (angiogenesis) in fracture healing (Li et al. 2003a; Koch et al. 1992) (Fig. 21.5, ph. 2).
Enhanced production of vascular endothelial growth factor (VEGF) by human mandibular osteoblasts, human peripheral blood monocytes (Doan et al. 1999) and human osteoblasts (hFOB1.19, MG-63 and SaOS-2) (Wang et al. 2004) has also been reported in response to LIPUS (Fig. 21.5, ph. 1). VEGF is a critical player in angiogenesis. It regulates mitogenesis and the recruitment of endothelial cells (Ferrara et al. 2003), and is also involved in osteoblast differentiation and osteoclast activation (Street et al. 2002; Ishiduka et al. 1999) (Fig. 21.5, ph. 2). Basic fibroblast growth factor (bFGF), another cytokine regulating angiogenesis in the healing fracture (Montesano et al. 1986; Hayek et al. 1987), has been found to be up-regulated by LIPUS treatment in human mandibular osteoblasts (Doan et al. 1999). bFGF enhances fracture healing through an unknown mechanism by promoting migration (Fig. 21.5, ph. 1) and mitogenesis of MSCs and osteoblasts (Radomsky et al. 1998; Kim et al. 2007; Globus et al. 1988) (Fig. 21.5, ph. 2).
Co-cultures of human osteoblast SaOS-2 and human umbilical vein endothelial cells exhibited higher levels of platelet-derived growth factor (PDGF) secretion upon LIPUS exposure (Ito et al. 2000; Kilian et al. 2004; Canalis et al. 1989). PDGF is a mitogenic factor for MSCs and osteoblasts (Ito et al. 2000; Kilian et al. 2004; Canalis et al. 1989) (Fig. 21.5, ph. 2).
21.5.1.3 NO and PGE2
Nitric oxide (NO), a free radical gas, was found to be an important contributor to bone formation in response to mechanical loading (Fox et al. 1996). The up-regulation of NO production in response to LIPUS was demonstrated to be involved in the regulation of VEGF expression in human osteoblasts (hFOB1.19) (Wang et al. 2004) (Fig. 21.5, ph. 1). The increase of NO production is in agreement with findings from Reher et al. (2002), also showing that NO concentration in human primary mandibular osteoblasts increases using both continuous and pulsed ultrasound systems. Moreover, they established that this increase was regulated by inducible NO synthase (iNOS) (Fig. 21.5, ph. 1).
Secretion of prostaglandin E2 (PGE2) has been shown to be up-regulated in response to ultrasound exposure in osteoblasts from various species (Tang et al. 2006; Reher et al. 2002; Sun et al. 2001; Naruse et al. 2003). PGE2 is an arachidonic acid-derived metabolite associated with bone formation and resorption (Nefussi and Baron 1985). Cyclooxygenase-2 (COX-2) is a rate-limiting enzyme in the PGE2 production reaction. It has been reported that LIPUS increases COX-2 gene expression in mouse pre-osteoblasts (Kokubu et al. 1999) (Fig. 21.5, ph. 1). In cells pretreated with a COX-2 specific inhibitor, PGE2 production in response to ultrasound was significantly decreased.
21.5.1.4 Early Osteogenesis
Insulin-like growth factor-1 (IGF-1) mRNA has been reported to be up-regulated by LIPUS in both the murine ST2 bone marrow derived cell line and rat osteoblasts within the first day of stimulation (Naruse et al. 2000, 2003) (Fig. 21.5, ph. 1). IGF-1 mediates expression of osterix (Osx), a transcription factor involved in differentiation of pre-osteoblasts into mature osteoblasts (Fig. 21.5, ph. 2) (Celil and Campbell 2005; Nakashima et al. 2002). The up-regulated expression of Osx has also been shown by Suzuki et al. (2009b) in a rat osteosarcoma-derived cell line expressing an osteoblastic phenotype, starting on day 7 of LIPUS exposure and persisting until the end of week 2 (Fig. 21.5, ph. 2, 3).
The up-regulation of early response gene c-fos following ultrasound treatment has been reported in rat MSCs, rat UMR-106 osteoblast-like cells, primary rat osteoblasts and murine ST2 cells (Naruse et al. 2000, 2003; Stein et al. 1996; Sena et al. 2005; Warden et al. 2001) (Fig. 21.5, ph. 1). Enhanced expression of c-jun, c-myc and Egr-1 genes in rat MSCs 3 h after 20 min LIPUS-stimulation has been demonstrated by Sena et al. (2005) (Fig. 21.5, ph. 1). The genes c-myc and Egr-1 encode for transcriptional factors involved in osteoblast proliferation and differentiation (Suva et al. 2013; Siggelkow et al. 1998; Kirstein and Baglioni 1988). The combination of c-jun and c-fos proteins forms an activator protein-1 (AP-1) transcriptional factor complex. AP-1 binding sites are encountered in several promoters that are important for the expression of osteogenic markers, i.e., collagen type 1 (Col-1) and osteocalcin (OC) (Bozec et al. 2010). The early up-regulation of these proteins, as well as other important osteogenic markers (e.g., osteonectin (ON), osteopontin (OPN), bone sialoprotein (BSP), alkaline phosphatase (ALP), bone morphogenetic protein 2 (BMP-2)), has been demonstrated in cells with an osteoblastic phenotype within 1 day following ultrasound exposure (Naruse et al. 2000, 2003; Gleizal et al. 2006; Yang et al. 2005; Maddi et al. 2006) (Fig. 21.5, ph. 1)
21.5.1.5 Proliferation of Osteoprogenitors and Osteoblasts
Proliferation of osteogenic progenitors is not directly affected by LIPUS exposure in-vitro as reported by Hasegawa et al. (2009). This group found that the number of human hematoma-derived progenitor cells remained the same within 7 days of stimulation, whereas differentiation of the cells was significantly enhanced within 28 days of LIPUS-exposure. LIPUS effects on osteoblast proliferation appear to be controversial. Doan et al. (1999) and Reher et al. (1998) demonstrated that DNA synthesis in human mandibular osteoblasts was increased 24 h post-exposure (Fig. 21.5, ph. 1). Hayton et al. (2005) showed an increase in gene expression of ornithine decarboxylase (ODC), a cell growth marker, 8 h following LIPUS-treatment in the human SaOS-2 osteosarcoma cell line (Fig. 21.5, ph. 2). These results are in agreement with a study by Wang et al. (2004) showing increased DNA synthesis 24-h post-ultrasound exposure in cultures of human osteoblasts hFOB1.19, MG-63 and SaOS-2. Several more studies reported an elevated proliferation rate in cells with osteoblastic phenotype within 4 days of LIPUS stimulation (Sant’Anna et al. 2005; Suzuki et al. 2009b; Gleizal et al. 2006; Li et al. 2003b; Hayton et al. 2005). In contrast to these findings, upon ultrasound-stimulation Suzuki et al. and Dalla-Bona et al. did not see any change in proliferation of rat ROS 17/2.8 osteosarcoma cells and the mouse OCCM-30 cementoblast cell line, respectively. Sawai et al. looked at proliferation of mouse MC3T3-E1 osteoblasts and osteosarcoma (mouse LM8 and human SaOS-2), renal cancer, prostate cancer and lung cancer cell lines. They found that it was not affected by 3 days of LIPUS stimulation, arguing for beneficial effects of ultrasound usage in patients with bone cancers and bone metastases. Increased proliferation of murine primary calvarial osteoblasts reported in Gleizal et al. (2006) study was accompanied by enhanced expression of osteogenic markers, i.e., ALP, BMP-2, BMP-7, OPN, Col-1 etc. Expression of these markers was normalized to expression of a housekeeping gene, compensating for a potential increased cell number. Therefore, it is not yet completely clear whether the beneficial effects of LIPUS concerning the fast and favorable healing of the in-vivo fracture are due to enhanced differentiation of osteoblasts, or to a more complex combination of accelerated proliferation, differentiation and maturation, finally leading to an increased tissue size.
21.5.1.6 Ossification
Another transcriptional factor with an essential role in the development of an osteogenic phenotype is Runx2. It has been reported that Runx2 can be up-regulated following ultrasound stimulation. Up-regulation can start from day 2 following ultrasound in the osteoblastic cell line ROS 17/2.8 and in rat MSCs (Suzuki et al. 2009b; Sant’Anna et al. 2005). Up-regulation can last up to 2 weeks following ultrasound in mouse calvarial primary osteoblasts (Sant’Anna et al. 2005; Suzuki et al. 2009b; Gleizal et al. 2006). Runx2 is encoded by the Cbfa1 gene (core-binding factor-1) and is only found in cells of osteoblast lineage (Ducy et al. 1999). Mice with Cbfa1−/− homozygous mutation are not capable of either intramembranous or endochondral ossification (Komori et al. 1997; Otto et al. 1997). Runx2 is a transcription factor regulating differentiation of MSCs into osteogenic lineage by directing expression of ALP, Col-1, matrix metalloproteinase-13 (MMP-13), BSP, OPN and OC genes (Ducy et al. 1999). Enhanced expressions of ALP and MMP13 have been observed on day 10, and elevated ALP activity was detected on day 6 in LIPUS-treated murine MC3T3-E1 cells (Unsworth et al. 2007) (Fig. 21.5, ph. 3). Up-regulated OPN and ALP expressions by ultrasound-exposure were also shown in osteoprogenitors and osteoblast-like cells (Sant’Anna et al. 2005; Naruse et al. 2000; Suzuki et al. 2009b; Gleizal et al. 2006; Unsworth et al. 2007; Maddi et al. 2006) (Imai et al. 2014). MSCs derived from adipose tissue also possess osteogenic potential, which can be enhanced by LIPUS stimulation in osteoinductive media (Yue et al. 2013). However, when the same cells were cultured in the media with adipogenic reagents, LIPUS supported adipogenic differentiation of the cells (Fu et al. 2013). In contrast to these results, it was shown that LIPUS could suppress the expression of phenotypic features of 3 T3-L1 adipocytes, down-regulate the expression of adipogenesis-related markers and up-regulate expression of osteogenesis-related markers in murine ST2 MSCs and MC3T3-E1 pre-osteoblasts (Kusuyama et al. 2014). These later results suggest that LIPUS stimulation suppresses adipogenesis and promotes osteogenesis of MSCs.
An effect of ultrasound on Dlx5 and Msx2 gene expression has been reported by Suzuki et al. (2009b). Increased gene expression of both Dlx5 and Msx2 was observed within the first 2 weeks of LIPUS treatment in rat osteoblast-like cells. The functional mechanisms of these proteins are not entirely understood. However, it is known that the Dlx5 gene is important for osteoblast differentiation and maturation, whereas Msx2 is in majority expressed during osteoblast proliferation and it antagonizes Runx2 (Ryoo et al. 1997). Dlx5 in turn binds to Msx2, therefore rescuing a prominent Runx2 transcriptional activity (Ryoo et al. 1997).
Physical immobility accompanying some bone fractures or other injuries can result in reduced rate of osteogenesis and induce adipogenesis of MSCs. However, when adipose tissue-derived hMSCs were placed in microgravity conditions, mimicking the lack of physical movement in humans, they experienced higher expression of Runx2, Osx, ALP and RANKL when treated with LIPUS. Conversely, they showed reduced expression of OPG, indicating a potential for LIPUS to restore normal osteogenic differentiation of MSCs from disuse by daily short duration stimulation (Uddin and Qin 2013).
21.5.1.7 BMPs
Bone morphogenetic proteins (BMPs), cytokines of the transforming growth factor-β (TGF- β) superfamily, perform a plethora of functions throughout diverse tissues, regulating cellular events based on the context (Ruschke et al. 2012).
BMP-2, 4 and 7 are important players in bone healing and regulate the differentiation of MSCs to the osteoblastic and chondroblastic lineages (Ahrens et al. 1993; Shen et al. 2010). BMPs signal through Smad, a family of transcription factors, and other cascades including mitogen-activated protein kinase (MAPK) pathways, like p38 and Erk1/2 (Heldin et al. 1997; Moustakas and Heldin 2005).
These proteins, as well as BMP receptors (BMPR-I, II) and activin receptors (ACVR- I, II), were found to be up-regulated after 7 days of LIPUS treatment in the rat osteosarcoma ROS 17/2.8 cell line (Suzuki et al. 2009a). Enhanced expression of BMP-2 from day 5 until day 14 has also been shown in these cells (Suzuki et al. 2009b). Smad1 activation, a signaling molecule downstream from BMPR receptors, was observed 5 min after LIPUS exposure.
21.5.1.8 Chondrogenesis
During the third stage of bone repair, proliferating MSCs start differentiating into chondrocytes and endochondral ossification begins (Fig. 21.5, ph. 3). Lee et al. (2006) reported that low intensity ultrasound delivered in a continuous wave mode promoted enhanced expression of chondrogenic markers Col-2, aggrecan (proteoglycan (PG)) and Sox-9 (a gene encoding for a transcriptional factor prominent for chondrocyte differentiation) at week 1 and 2 in rabbit MSCs (Fig. 21.5, ph. 3). Schumann et al. (2006) demonstrated that hMSCs seeded in 3D scaffold and stimulated with 40 min a day LIPUS for 1 week, and then left in the incubator for another 2 weeks, have a more pronounced chondrogenic phenotype on day 21, expressing higher aggrecan, Col-1, Col-2 and Col-10 (Fig. 21.5, ph. 4). Other studies showed that LIPUS supported differentiation of rat and pig primary chondrocytes within the first 2 weeks of the treatment and up–regulated expressions of TGF-β, TGF-β R1, PG, Col-2 and Col-9 (Mukai et al. 2005; Takeuchi et al. 2008; Kobayashi et al. 2009) (Fig. 21.5, ph. 3). Parvizi et al. (2002) reported that proteoglycan increase in rat chondrocytes treated by ultrasound was governed by increase of intracellular Ca2+ concentration.
Besides their differentiation state, viability of chondrocytes was also affected by LIPUS. Several studies have shown that proliferation of chondrocytes from rat, pig and the human nucleus pulposus cell line (HNPSV-1) was accelerated within 2 weeks of LIPUS treatment (Mukai et al. 2005; Takeuchi et al. 2008; Kobayashi et al. 2009) (Fig. 21.5, ph. 3).
LIPUS is a potential candidate for cartilage repair in patients suffering from osteoarthritis (OA). It has been shown that LIPUS has a beneficial effect on the migratory ability of bovine chondrogenic progenitor cells, which were recruited by ultrasound to the mechanically damaged cartilage through focal adhesion kinase (FAK) activation (Jang et al. 2014). Rabbits with anterior cruciate ligament transection have been used as a model for OA. It was found that chondrocytes from this model produced higher amounts of Col-2 and aggrecan at both the mRNA and protein level when stimulated with LIPUS, whereas MMP-1 and MMP-13 levels were reduced after the ultrasound application (Cheng et al. 2014).
21.5.1.9 Bone Remodeling
At the primary spongiosa level, chondrocytes become hypertrophic and begin to secrete ALP, which initiates matrix mineralization. The mineralized cartilage matrix provides a scaffold for migration of osteoprogenitor cells, which then differentiate and produce osteoid, the organic matrix of mineralized bone tissue (Fig. 21.5, ph. 4). Enhanced expression of maturating osteoblast markers, like OC and BSP, and calcium deposition in response to ultrasound has been confirmed throughout several studies (Yang et al. 2005; Suzuki et al. 2009b; Unsworth et al. 2007; Angle et al. 2011; Dalla-Bona et al. 2006; Ren et al. 2013). Osteoblast receptor activator of NF-κB ligand (RANKL) and osteoprotegerin (OPG) protein expression is responsible for regulation of osteoclast function. While RANKL activates osteoclasts (Lacey et al. 1998), OPG antagonizes their action by binding to the receptor activator of NF-κB (RANK) (Hsu et al. 1999). The most pronounced peak (about tenfold compared to unstimulated controls) of RANKL gene expression was found during the third week of LIPUS-treatment in differentiating murine osteoblasts, while it was only slightly up-regulated during 1st, 2nd and 4th week of stimulation. Conversely, OPG expression levels remained constant throughout 3 weeks of LIPUS-stimulation (Bandow et al. 2007). These results imply that LIPUS enhances osteoclastogenesis throughout the entire time-course of bone regeneration (Fig. 21.5, ph. 2, 3, 4), peaking at week 3, which corresponds to woven bone resorption and lamellar bone formation in mice.
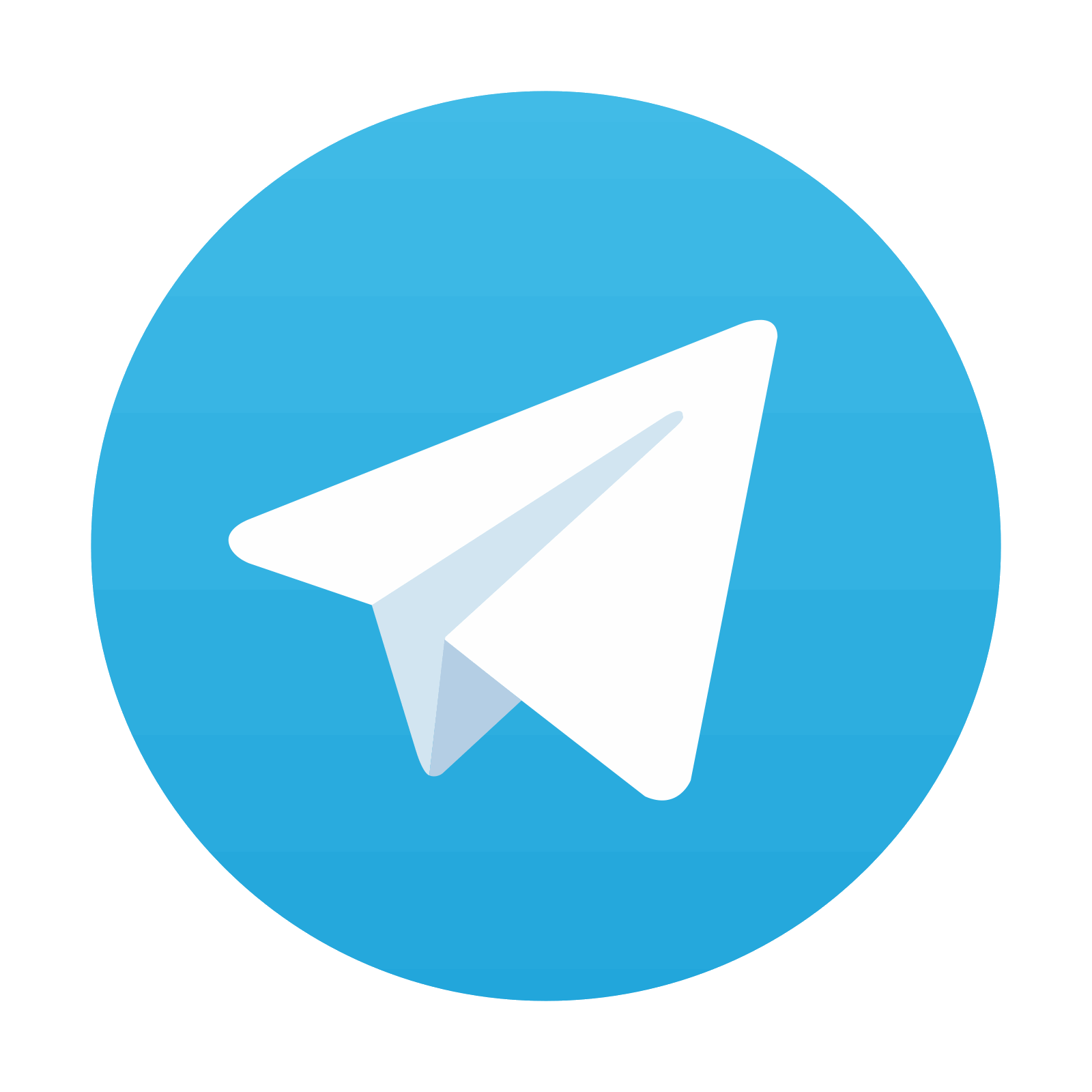
Stay updated, free articles. Join our Telegram channel
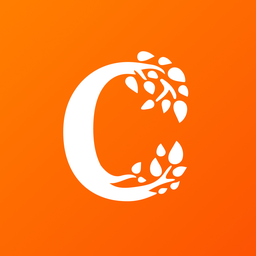
Full access? Get Clinical Tree
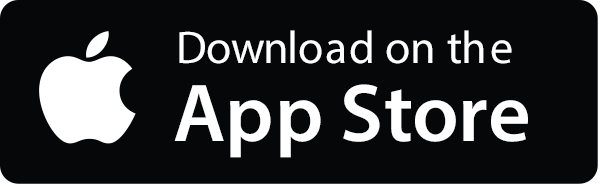
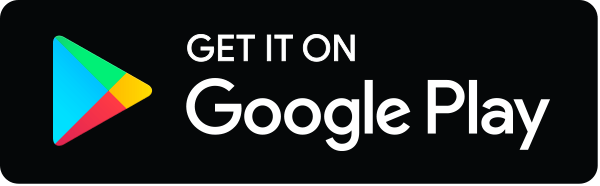