Willi A. Kalender
3.2.1 Introduction
X-ray CT has been in clinical use since 1972, though it made only minor contributions to cardiac imaging during its first two decades of existence. The scan times were too long to deliver reliable results that could be incorporated into clinical routines. The same was true of MRI, the other tomographic imaging procedure available at that time.
The 1990s, however, brought rapid technological development that continues to this day. The fact that the newest modern technologies can depict the entire heart in less than 1 second with an effective slice scan time of under 100 ms can be attributed to the early development of electron beam CT. This technique of electron beam CT allowed cardiac imaging with a temporal resolution of 50 ms, albeit with limited spatial resolution. In particular, the development of helical CT with the subsequent significant increase in rotational velocity, the development of
multiple-row detector systems, and DSCT (dual source CT) were the primary contributors to cardiac CT as it is performed today.
Accordingly, cardiac CT possesses great potential, especially for the noninvasive visualization of the coronary arteries. To date, it has rarely been used on children with congenital heart defects due to high radiation exposure. The different sections in this chapter will depict and discuss the following subjects: modern CT technology, special technological solutions for CT images of the heart and the resultant image quality, aspects of patient dose and radiation protection, and, finally, recommendations for scan techniques and dose optimization during pediatric cardiac exams.
3.2.2 Modern Computed Tomography Technology
The development of the CT technique and the underlying principles has already been discussed in many publications, including those by Kalender.
31 Therefore, this section will provide only a brief discussion of the newest developments relevant to cardiac CT in cases of congenital heart defects. Since 1989, the basis of modern CT has been the
spiral scan procedure.
32 It is based on modern slip ring technology—whereby the detector and tubes continually rotate around the patient—and has been implemented in all modern CT scanners for more than a decade. Helical CT allows very rapid, continual scanning of the patient with no gaps along the body’s longitudinal axis and, depending on patient compliance, virtually no respiratory artifacts.
The development of multiple-row detectors was another extremely significant step in the development of helical CT: this technology, which allowed four slices to be acquired at once, was made available simultaneously by virtually all manufacturers in 1998. Rapid further development has been noted since then, such as the 16-slice scanner in 2001, the 64-slice scanner in 2004, and numerous other detectors that have been placed on the market since then. At times, this was referred to as the “slice race,” since development seemed to continue more or less independently of clinical requirements.
A further technological development meant specifically to support cardiac imaging was
DSCT, namely scanners that use two complete measurement systems, meaning two X-ray tubes and two detectors (▶
Fig. 3.27).
31,
33 Both measurement systems are placed at the same level and attached rigidly to one another so that the data from both systems can be combined and contribute jointly to image generation. Devices designed in this fashion offer decisive advantages: the combination of data measured by both
systems allows the effective scan time to be cut in half. At minimum, a scan range of 180° plus fan angle is needed for image reconstruction. DSCT scans can provide this information after only a 90° rotation. Furthermore, double X-ray power is available. This is of great importance particularly to images with short effective scan times, since this exposes the patient to the required radiation dosage for the shortest possible period of time.
The most important
scanner and image quality parameters are summarized in ▶
Table 3.3. Nowadays, modern scanners offer rotation times of 0.27-0.35 seconds for a full 360° rotation. This means that for partial scans, the minimum acquisition time per image is 140-200 ms for simple scanners, but only 75-85 ms for DSCT. The minimum slice thickness is 0.500-0.625 mm, which also yields high 3-D resolution (meaning along the body’s longitudinal axis) in all multiplanar and 3-D views. This ensures high, isotropic local resolution; in this context,
isotropic means that local resolution is approximately the same in all three spatial dimensions, and thus that the examined volume can be viewed and evaluated 3-dimensionally from any orientation. This is of particular importance when examining the coronary arteries, for example. Resolution may be less than 1 mm, but isotropic resolutions of 0.5 mm can be achieved routinely.
Image quality is generally good (▶
Fig. 3.28), and increased acquisition speed does not generally correlate with reduced image quality.
34 Another positive effect of increased acquisition speed is that the patient dosage is reduced significantly due to increased table shift. This will be discussed in greater detail later in this chapter.
3.2.3 Technical Approaches to Cardiac Imaging
The main goal of cardiac imaging is to depict the anatomy without blurring or imaging artifacts caused by movement. Thus, the effective scan time must be significantly shorter than the length of a cardiac cycle. Furthermore, it is desirable that the scan time, typically indicated in % of the contraction cycle, can be predetermined and selected freely based on cardiac phase. Precise assignment to a cardiac phase is important for diagnostic purposes, e.g., if it is necessary to depict the heart over the entire cardiac cycle as a 4-D data set for functional analysis, or if the data needs to be collected for the phase of the cardiac cycle involving the least movement—namely end diastole. For this reason, it is generally necessary to record an ECG simultaneously with all image acquisitions of the heart in order to achieve a depiction with few movement artifacts. Two different procedures—prospective ECG triggering and retrospective ECG gating—are implemented in order to ensure assignment to a cardiac phase. In the former, measurements generally begin during diastole, based on the desired ECG time. In the latter, continually acquired CT data is assigned retrospectively to the ECG data collected in parallel in order to reconstruct images.
In principle, prospective ECG triggering is easier to perform and also generally means a lower dose for the patient, since the patient is only exposed to radiation during the desired cardiac phase. Prospective triggering, however, can fundamentally only be performed in cases of low heart rate, and thus frequently cannot be used in children with high heart rates. When taking contraindications into account, it is occasionally possible to administer a beta blocker to reduce heart rate. The movement of the heart or valves cannot, however, be evaluated via prospective triggering.
Retrospective ECG gating procedures, on the other hand, still allow imaging data to be acquired after reconstruction, and thus at the optimal time, thereby increasing the likelihood of good image quality. Furthermore, retrospective procedures allow the reconstruction of temporally resolved 4-D exams—such as exams of the heart’s valves or the ventricles over the entire cardiac cycle—which can then be observed in motion. Dynamic exams of this type have been performed successfully for years via spiral CT with retrospective gating, though this is associated with a higher radiation exposure than prospective triggering. Only a very low table shift or pitch is possible for retrospective gating, since data must be collected for every cardiac phase.
31
For retrospective gating, scans are performed with a pitch of 0.2-0.4, which means overlapping data acquisition and thus generally a higher patient dose. In order to reduce dose, prospectively triggered single scans have been performed sequentially (“step and shoot”) over the past few years, corresponding to a pitch of 1 and thus a lower dose. These scans cannot, however, depict any motion over time, which is necessary for functional analysis.
Broad detector scanners can depict a child’s entire heart in a single rotation and are thus especially attractive, particularly for dynamic exams. For example, a device that offers 320 slices at 0.5 mm each can image approximately 12 cm during a single rotation. While this is not always adequate to depict a full adult heart, it generally suffices for small children. One current drawback of these scanners is their somewhat lower temporal resolution.
DSCT can be implemented with a pitch greater than 3 and/or in conjunction with prospective triggering (▶
Fig. 3.28). If high-pitch mode can be implemented, this results in a very high temporal resolution of circa 75 ms, a very short overall scan time of approximately 0.25 seconds (meaning acquisition during a single heartbeat), and a low dose of circa 1 mSv (▶
Fig. 3.29). Though high-pitch mode can only be used with a low heart rate, image quality corresponds to that during normal operation.
34
3.2.4 Patient Dose and Radiation Protection
We cannot and should not go into great detail in this book regarding the technical and physical aspects of dosimetry in CT. This information is discussed in depth in the literature in this field.
31,
35,
36
The most significant variables are the CT dose index and the dose-length product, which nowadays are displayed on the operation console of every modern CT scanner and, according to the German X-Ray ordinance, must also be documented. The CT dose index is a value specific to each device and scan mode (independent of tube voltage and slice thickness) that is specified by the manufacturer and must be recorded during quality inspection. Constancy tests for the CT dose index performed regularly by technical personnel and/or a medical physicist (generally at least twice per year) ensure that the patient dose does not change. The CT dose index is determined using 10-cm-long ionization chambers in Acrylic Glass phantoms (cylinders made of polymethyl methacrylate with diameters of 16 and 32 cm for skull or thoracic exams, respectively). The CT dose index measured on the phantoms without table shift must then be corrected by the pitch value p for the respective patient exam. The volume CT dose index is calculated by division:
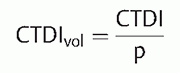
whereby
CTDIvol = volume CT dose index
CTDI = CT dose index
p = pitch.
The volume CT dose index, which is indicated using a dose unit of Gy or mGy, is not used to estimate the patient’s radiation exposure. Rather, this index is only used to document that the device is functioning properly and that the correct exam parameters were chosen for the respective patient exam. The volume CT dose index does, however, allow an initial estimate of local dose (e.g., organ doses in the examined area), provided that the patient data is interpreted correctly (patient thickness compared to the Acrylic Glass phantom).
The dose–length product, on the other hand, provides values that allow an initial, preliminary estimate of the patient’s radiation exposure (effective dose). An automatic recording is created for each CT exam specifically for the implemented CT protocol and the selected scan parameters. This recording is then displayed on the CT scanner’s operating panel. The following formula is used:
where
DLP = dose-length product
CTDI = CT dose index
C = tube current-time product
N = number of rotations
M = number of slices
S = slice thickness.
100 = measured over a 100 mm long ionization chamber
w = weighted
i = tissue weighting factor
In essence, this formula means that to calculate the dose-length product, the standardized CTDI value must be multiplied by the mAs value for the tube current-time product of the respective exam scan and the length of the examined area (slice thickness multiplied by the number of slices and rotations). For repeated exams, the product must be added up for all exam indices (index i). This results in values using the unit mGy × cm, which, like the values for the dose-area product in projection radiography, are a measure of radiation exposure, and can be referenced to compare exams in the same section of the body.
Thus, the dose-length product, in addition to the volume CT dose index, is also a basis for defining
diagnostic reference dose values. These diagnostic reference dose values have been legally binding in the European Union since 2000 and in Germany since 2002, with the amended X-Ray Ordinance. The diagnostic reference dose values are stipulated by law in Germany by the Federal Agency for Radiation Protection
37 and are adapted to the most up-to-date technological methods every few years. Specifying diagnostic reference dose values provides the examiner with comparative values for optimizing his scan parameters for patient dose via benchmarking. The diagnostic reference dose values are determined by the Federal Agency for Radiation Protection, based on national surveys. Thus, a diagnostic reference dose value for a dose size for a specific exam type is compared to the value for the third quartile (75th percentile) while distributing mean value for the individually recorded institutions. This means that, based on specifications, circa 25% of German institutions that perform this exam need to lower their values (for CT, the volume CT dose index, and the dose-length product). Long-established medical authorities regularly check the dose value to be set by the examiner compared to diagnostic reference dose values on a regular basis, in addition to justified indication, adequate image quality, and correct findings. If the statistical mean dose value exceeds the diagnostic reference dose value by more than 30%, supervisory authorities may be informed due to unjustifiably high
exam doses. The medical authority will, however, first attempt to consult with examiners to reduce median dose values.
The actual dose unit relevant to patient risk is the
effective dose. This dose can generally be estimated using the Monte Carlo method for average people (average man: 70 kg, 170 cm tall) based on information from the dose-length product.
35,
38 This procedure is established, and the corresponding 3-D distribution of patient dose can be seen in ▶
Fig. 3.29. These distributions determine the dose for individual organs in the direct path of radiation as well as all other organs exposed to radiation. The effective dose is calculated as a weighted median of the relative sensitivity of the individual organs from the whole of organ dose values.
31,
36 This procedure, which estimates effective dose in this manner using the dose-length product, is established
35 and is an approach for estimating doses in a manner appropriate for daily practice.
Procedures for evaluating
CT doses in children are not defined and standardized to the same extent as those for CT in adults. The information for volume CT dose indices and dose-length products cannot be easily applied to pediatric exams. The skull and thoracic thicknesses of 16 and 32 cm phantom diameters, respectively (which are used for estimating doses in adults) do not adequately represent the anatomical proportions in children. The common conversion values from the dose-length product for effective dose calculations as listed in the literature should be evaluated critically. To date, the Federal Agency for Radiation Protection has made no statements on this matter. The American Association of Physicists in Medicine provides recommendations for using conversion factors,
39 which were also taken into account in ▶
Table 3.4. A direct dose calculation based on concrete patient profiles and organ dose values (thus providing an estimate of effective dose) would be more precise (▶
Fig. 3.29), though this is not always feasible in everyday practice. Since 2010, it has been recommended that, at minimum, CT manufacturers refer to the smaller Acrylic glass phantom diameter of 16 cm value for the volume CT dose index and the dose-length product shown on the CT during pediatric exams. Special phantom diameters for pediatric CT protocols, a topic of discussion in recent years, have not yet been introduced. It is expected, however, that the Federal Agency for Radiation Protection will soon publish graded reference dose values for pediatric CT exams for children in various age groups. An approach based on size with standard phantoms for all age groups, similar to the procedure for adults (▶
Fig. 3.30), might offer additional benefits.
38