Functional neurosurgery involves precise surgical targeting of anatomic structures to modulate neurologic function. From its conception, advances in the surgical treatment of movement disorders have been intertwined with developments in medical imaging, culminating in the use of stereotactic magnetic resonance imaging (MRI). Meticulous attention to detail during image acquisition, direct anatomic localization, and planning of the initial surgical trajectory allows the surgeon to reach the desired anatomic and functional target with the initial trajectory in most cases, thus reducing the need for multiple passes through the brain, and the associated risk of hemorrhage and functional deficit. This philosophy is of paramount importance in a procedure that is primarily aimed at improving quality of life. Documentation of electrode contact location by means of stereotactic imaging is essential to audit surgical targeting accuracy and to further the knowledge of structure-to-function relationships within the human brain.
Functional neurosurgery
Functional neurosurgery involves precise surgical targeting of anatomic structures to modulate neurologic function. The ultimate aim is to improve the symptoms and quality of life of patients suffering from chronic neurologic disorders; this demands minimal risk of inflicting morbidity and mortality. Well-established indications for functional neurosurgery include movement disorders such as Parkinson disease (PD), several forms of dystonia, essential tremor, and chronic pain. Other indications proposed in recent literature, either novel or revisited, are less well established but hold promise for patients with chronic debilitating conditions including cluster headache, Tourette syndrome, obsessive compulsive disorder, severe refractory depression, and epilepsy. This article focuses on the role of imaging in the surgical treatment of movement disorders.
Imaging has played a central role in the practice of functional neurosurgery since its inception. The current situation and likely future directions are best appreciated by reviewing their parallel development.
The early years of functional neurosurgery
Initial attempts to surgically control abnormal movements involved drastic measures. Functional neurosurgery originated in the late nineteenth century when Victor Horsley extirpated large areas of motor cortex for the relief of athetosis. Desperate assaults on the pyramidal system, at cranial and spinal levels, were invariably accompanied by significant motor deficit. Meyers suggested the basal ganglia as a target for surgery for involuntary movement disorders in the following decades, and in the 1940s the target for ablation moved to the so-called extrapyramidal system. Although these adventurous, open procedures proved that effective surgery need not impose significant neurologic deficit, they were associated with prohibitive mortality rates.
Cooper refocused attention on the basal ganglia as a target for surgical interventions when he noted the beneficial effect on contralateral tremor after accidentally ligating the anterior choroidal artery (AChA) in a patient with PD. The variable vascular territory of the AChA makes this an inconsistent way of performing basal ganglia lesions, but the incident highlighted the pallidum and thalamus as possible targets in PD.
The inconsistent results and high risk of vascular ablation pressed surgeons to develop alternative approaches. Probes were placed deep within the brain parenchyma via a burr hole under local anesthetic, initially guided solely by plain radiography and surgeon’s intuition for anatomic localization. Reversible lesions with local anesthetic or mild thermal manipulation allowed the effect of the intervention to be assessed before permanent chemical or thermal ablation. Despite functional improvements in individual patients, uncertainty about the anatomic location of the lesion interfered with replication of clinical results. Clearly, a more reliable means of navigation within the brain was required.
The Stereotactic Technique
Precise neurosurgical targeting relies on the stereotactic technique, the principles of which were established by Clarke and Horsley in 1908. “Stereotactic” literally means “three-dimensional touch,” which conveys the philosophy behind this powerful navigational tool. It provides accurate localization of an intracranial target by ascertaining its triplanar coordinates with reference to fixed landmarks, and provides surgical access to targets deep within the brain in a minimally invasive fashion.
Historical inability to visualize the desired intracranial target led to the development of stereotactic atlases depicting the spatial relations of neural anatomy with respect to visible landmarks. At the beginning of the twentieth century, medical imaging technology was limited to Roentgen’s development of radiographs in 1895. The first stereotactic atlases therefore defined the spatial relations of cerebral structures with reference to bony landmarks. Considerable variability between bony cranial landmarks and enclosed neural structures severely limited the accuracy of such anatomic targeting.
Ventriculography and Its Legacy
The limited value of plain radiography in the localization of even large intracranial lesions drove Walter Dandy to introduce air as a contrast medium outlining the ventricles as described in his seminal article of 1918.
Spiegel and colleagues combined stereotactic principles with ventriculography to introduce the stereotactic technique to surgical practice in 1947. A frame applied to the skull provides visible external reference points to intracranial coordinates. The coordinates of intracerebral landmarks, such as the anterior and posterior commissures (AC, PC), are acquired by imaging the patient’s brain with the frame in situ. These neural landmarks enjoyed a much tighter spatial relation to the basal ganglia than did the bony landmarks of the cranium. Stereotactic atlases based on such neural landmarks therefore provide a more accurate method of anatomic localization, but do not eliminate the problem of anatomic variability completely. Nevertheless, the AC PC line still plays a central role in modern functional neurosurgical practice. In the 1950s and 1960s, the combination of the stereotactic technique, stereotactic atlases, and ventriculography allowed safe targeting of deep-seated structures, and the discipline of functional neurosurgery flourished. Tens of thousands of patient with psychiatric and neurologic indications underwent functional neurosurgical operations in the years that followed. Cooper’s service alone conducted almost 7000 functional interventions over 15 years.
Refining of the Anatomic Target
Functional neurosurgical interventions are traditionally performed under local anesthesia. This technique allows surgeons to assess the clinical effects of the surgical intervention in “real time,” in terms of the positive effect on symptoms and possible undesirable side effects. Diverse methods may be used to produce “reversible” lesions of the target area to assess the clinical effects, including thermal manipulation, injection of local anesthetic and high frequency electrical stimulation. Physiologic observations during surgery include measurement of electrical impedance and recording of neural activity (via micro- or macroelectrodes). These data allow functional neurosurgeons to corroborate the anatomic location of their surgical probe with physiologic and clinical findings.
Computerized Tomography and the Functional Hiatus
Functional surgery for motor disorders had become well established by the mid-1960s, but the number of procedures performed plummeted after the introduction of L-DOPA for the treatment of PD in 1967. The introduction of pharmacologic means of treating psychiatric disorders, combined with the public backlash against flagrant abuse of surgical interventions by a few notorious but highly active individuals, led to the effective demise of psychiatric surgery. Computerized tomography (CT) was introduced in the 1970s following the work of Hounsfield, and allowed visualization of the AC and PC in a noninvasive fashion. However, the subspecialty field of functional neurosurgery had virtually come to a halt.
The early years of functional neurosurgery
Initial attempts to surgically control abnormal movements involved drastic measures. Functional neurosurgery originated in the late nineteenth century when Victor Horsley extirpated large areas of motor cortex for the relief of athetosis. Desperate assaults on the pyramidal system, at cranial and spinal levels, were invariably accompanied by significant motor deficit. Meyers suggested the basal ganglia as a target for surgery for involuntary movement disorders in the following decades, and in the 1940s the target for ablation moved to the so-called extrapyramidal system. Although these adventurous, open procedures proved that effective surgery need not impose significant neurologic deficit, they were associated with prohibitive mortality rates.
Cooper refocused attention on the basal ganglia as a target for surgical interventions when he noted the beneficial effect on contralateral tremor after accidentally ligating the anterior choroidal artery (AChA) in a patient with PD. The variable vascular territory of the AChA makes this an inconsistent way of performing basal ganglia lesions, but the incident highlighted the pallidum and thalamus as possible targets in PD.
The inconsistent results and high risk of vascular ablation pressed surgeons to develop alternative approaches. Probes were placed deep within the brain parenchyma via a burr hole under local anesthetic, initially guided solely by plain radiography and surgeon’s intuition for anatomic localization. Reversible lesions with local anesthetic or mild thermal manipulation allowed the effect of the intervention to be assessed before permanent chemical or thermal ablation. Despite functional improvements in individual patients, uncertainty about the anatomic location of the lesion interfered with replication of clinical results. Clearly, a more reliable means of navigation within the brain was required.
The Stereotactic Technique
Precise neurosurgical targeting relies on the stereotactic technique, the principles of which were established by Clarke and Horsley in 1908. “Stereotactic” literally means “three-dimensional touch,” which conveys the philosophy behind this powerful navigational tool. It provides accurate localization of an intracranial target by ascertaining its triplanar coordinates with reference to fixed landmarks, and provides surgical access to targets deep within the brain in a minimally invasive fashion.
Historical inability to visualize the desired intracranial target led to the development of stereotactic atlases depicting the spatial relations of neural anatomy with respect to visible landmarks. At the beginning of the twentieth century, medical imaging technology was limited to Roentgen’s development of radiographs in 1895. The first stereotactic atlases therefore defined the spatial relations of cerebral structures with reference to bony landmarks. Considerable variability between bony cranial landmarks and enclosed neural structures severely limited the accuracy of such anatomic targeting.
Ventriculography and Its Legacy
The limited value of plain radiography in the localization of even large intracranial lesions drove Walter Dandy to introduce air as a contrast medium outlining the ventricles as described in his seminal article of 1918.
Spiegel and colleagues combined stereotactic principles with ventriculography to introduce the stereotactic technique to surgical practice in 1947. A frame applied to the skull provides visible external reference points to intracranial coordinates. The coordinates of intracerebral landmarks, such as the anterior and posterior commissures (AC, PC), are acquired by imaging the patient’s brain with the frame in situ. These neural landmarks enjoyed a much tighter spatial relation to the basal ganglia than did the bony landmarks of the cranium. Stereotactic atlases based on such neural landmarks therefore provide a more accurate method of anatomic localization, but do not eliminate the problem of anatomic variability completely. Nevertheless, the AC PC line still plays a central role in modern functional neurosurgical practice. In the 1950s and 1960s, the combination of the stereotactic technique, stereotactic atlases, and ventriculography allowed safe targeting of deep-seated structures, and the discipline of functional neurosurgery flourished. Tens of thousands of patient with psychiatric and neurologic indications underwent functional neurosurgical operations in the years that followed. Cooper’s service alone conducted almost 7000 functional interventions over 15 years.
Refining of the Anatomic Target
Functional neurosurgical interventions are traditionally performed under local anesthesia. This technique allows surgeons to assess the clinical effects of the surgical intervention in “real time,” in terms of the positive effect on symptoms and possible undesirable side effects. Diverse methods may be used to produce “reversible” lesions of the target area to assess the clinical effects, including thermal manipulation, injection of local anesthetic and high frequency electrical stimulation. Physiologic observations during surgery include measurement of electrical impedance and recording of neural activity (via micro- or macroelectrodes). These data allow functional neurosurgeons to corroborate the anatomic location of their surgical probe with physiologic and clinical findings.
Computerized Tomography and the Functional Hiatus
Functional surgery for motor disorders had become well established by the mid-1960s, but the number of procedures performed plummeted after the introduction of L-DOPA for the treatment of PD in 1967. The introduction of pharmacologic means of treating psychiatric disorders, combined with the public backlash against flagrant abuse of surgical interventions by a few notorious but highly active individuals, led to the effective demise of psychiatric surgery. Computerized tomography (CT) was introduced in the 1970s following the work of Hounsfield, and allowed visualization of the AC and PC in a noninvasive fashion. However, the subspecialty field of functional neurosurgery had virtually come to a halt.
Resurgence of functional neurosurgery
Radiofrequency Thermal Lesions
During the last 2 decades of the twentieth century it became increasingly clear that medication was not the panacea it was initially believed to have been. Drug resistant symptoms, tolerance, and side effects led surgeons to re-explore the surgical approaches of earlier years. Laitinen’s seminal article revisiting Leksell’s posteroventral radiofrequency pallidotomy was an important factor in this resurgence. Radiofrequency thermal lesions remain an excellent surgical technique offering a cheap and effective means of controlling the symptoms of various movement disorders at numerous brain targets. The increased risk of adverse effects with bilateral basal ganglia lesions and their permanent nature have led to a decline in the use of this technique in favor of more modern alternatives.
New Technologies: Deep Brain Stimulation and Magnetic Resonance Imaging
Exploration of neural activity in the cortex and basal ganglia in health and disease suggested possible new targets for intervention, such as the subthalamic nucleus (STN) in PD, popularized by Benabid’s group. Fears of causing intolerable side effects provided the drive to commercially manufacture implantable hardware necessary for chronic high frequency stimulation of the brain. Deep brain stimulation (DBS) has a similar functional effect to lesions but allows greater reversibility, flexibility, and adaptability than a permanent lesion. The most commonly used device for chronic DBS uses an implanted subclavian impulse generator (IPG) delivering variable monopolar or bipolar stimulation through any combination of 4 contacts at the end of a quadripolar electrode ( Fig. 1 ).
Advances in imaging have had an immense impact on every branch of neurosurgery, and functional neurosurgery is no exception. With the resurgence of functional procedures, a few surgeons returned to the surgical approach of earlier years, relying on ventriculography and stereotactic atlases for their initial targeting. However, many surgeons turned toward the technological advances in axial imaging for initial anatomic targeting. Leksell, with characteristic insight, recognized the fundamental importance of magnetic resonance imaging (MRI) in the practice of functional neurosurgery: “In clinical practice, brain imaging can now be divided in two parts: the diagnostic neuroradiology and the preoperative stereotactic localization procedure. The latter is part of the therapeutic procedure. It is the surgeon’s responsibility and should be closely integrated with the operation.” The accuracy, speed and noninvasive nature of MRI compared with ventriculography is a major factor in its increasing use as the primary tool for anatomic targeting by functional neurosurgeons. Nevertheless, several obstacles needed to be addressed before these advantages could be applied to clinical practice.
Structural Imaging
The tissue contrast visible within the brain parenchyma on early MR images was superior to that of CT but poor by modern standards. This limitation was rapidly addressed with advancing MR technology, higher field strengths, newer head coils, improved signal-to-noise ratios, and the introduction of a multitude of MR sequences that could be tailored to the area of interest.
The imaging protocols used in functional neurosurgery must establish a compromise between various factors. Target structures are often small, placing demands on image resolution. Visualization requires high tissue contrast between the target structure and the surrounding tissues. There is a limit to how long patients undergoing awake surgery can keep still in the MRI scanner, which places practical limits on image acquisition time. Acquired images should be contiguous thin slices. A voxel size of 1 × 1 × 2 mm is often seen as an acceptable compromise between image resolution, signal-to-noise ratio, and practical image acquisition time on 1.5 T scanners. The publication of various imaging protocols that meet these criteria for specific anatomic targets allows for direct visualization on stereotactic MRI before surgical targeting (see later discussion).
Unlike diagnostic radiology, imaging for stereotactic surgery requires more than just visualization of structures. Inevitable inhomogeneities of the magnetic field can lead to geometric distortion of the acquired images. This represents a significant obstacle to accurate spatial representation and can render such MR images useless for the purpose of accurate anatomic targeting ( Fig. 2 ).
An increasingly popular approach is to address the issue of MR distortion directly. Several methods for correction of field inhomogeneities have been described. Manufacturers of MR scanners have incorporated software solutions that correct for distortion, resulting in a greatly improved geometric accuracy of MR images. With adequate care and quality control, geometric errors can be reduced to the order of the pixel size and the submillimetre range.
The use of stereotactic MRI in functional neurosurgery has practical implications for the design, structure, and application of the stereotactic frame. Evidently, the frame must be MR-compatible so that it exerts minimal distortion of the MR field. Nevertheless, minimal geometric distortion can still be an issue close to the base of the frame. The surgeon should bear this in mind when applying the frame to the head such that the frame is as far away from the target region as possible. Coronal images may suffer from greater distortion than axial images because the lower fiducials that lie close to the frame are required for stereotactic calculation on coronal images. It is therefore recommended that axial images are used in preference to coronal images when working with stereotactic MR images.
Geometric accuracy at the center of the MRI field tends to be excellent; however, distortion is exacerbated at the field periphery. Therefore, frames with smaller fiducial indicator boxes (such as the Laitinen or Leksell systems) enjoy less geometric distortion than their larger counterparts. When preparing the patient for imaging, the surgeon should ensure that the radiographer advances the region of interest to the center of the magnetic field, as this tends to improve the geometric accuracy of the acquired images. With adequate quality control it is becoming clear that MRI distortion need not be a clinically significant issue when used for anatomic targeting in functional neurosurgery.
Another approach attempts to circumvent such problems by fusing or morphing nonstereotactic MR data onto stereotactic CT images, thus benefiting from contrast-rich MR data at the center of the field and the geometrically accurate fiducial localization of CT at the field periphery. Numerous commercially available software packages now allow for this facility. However, this method remains prone to errors of image fusion that may ultimately dwarf the spatial errors introduced by MRI distortion.
Newer MR technologies result in fresh challenges: the faster and stronger gradient systems, shorter-bore magnets, and higher field strengths popular in modern magnets further compromise field homogeneity; however, awareness of the problem has led to innovative solutions. Functional neurosurgery is renowned for its multidisciplinary nature, with excellent results being fostered in centers that enjoy close collaboration between neurologist and neurosurgeon. There is no doubt that in the modern era, the MR physicist and neuroradiologist are key players in the multidisciplinary approach to functional neurosurgery. Imaging of the most popular and promising brain targets used in the surgical treatment of movement disorders is reviewed below.
Indirect Localization
Recognizing the AC and PC is an important step in indirect targeting. Once the AC, PC, and midcommissural points are defined in space, standard coordinates derived from stereotactic atlases can provide the surgeon with an estimated location of the target structure. Wherever possible, direct MRI visualization can then be used to refine the anatomic target.
The AC is a compact bundle of white matter connecting the olfactory and temporal regions of the 2 hemispheres. Lying in the anterior wall of the third ventricle, 4 to 6 mm inferior to the foramen of Munro, it is often present on more than 1 axial image when contiguous 2-mm slices are acquired. Historically, it was the most posterior border of the AC that could be seen on ventriculography, and it is this point that should be defined on MR imaging ( Fig. 3 ). The PC lies at the posterior aspect of the third ventricle and connects nuclei involved in eye movements and the pupillary light reflex. On axial images, the third ventricle will be noted to have a box shape posteriorly. As one reaches the aqueduct, this box shape of the third ventricle disappears to give way to a funnel shape. The PC lies immediately rostral to the aqueduct and, on contiguous images, is therefore present on the most inferior axial slice before this narrowing occurs (see Fig. 3 ). The AC and PC are best defined on T1-weighted MR images. The PC may occasionally be difficult to discern, especially on CT images of younger patients with narrow third ventricles.
Standard coordinates give an estimate of the location of surgically relevant targets in relation to the midcommissural point. Anatomic variability results in diverse coordinates being quoted for the same structures. The present author uses the following coordinates from the midcommissural point for initial indirect localization and refines these further by direct visualization of the target structure wherever possible: STN: 12 mm lateral, 2 mm posterior, 5 mm inferior; posteroventral pallidum: 21 mm lateral, 2 mm anterior, 5mm inferior; motor thalamus: 13 to 15 mm lateral, 6 mm posterior, at the level of the AC PC plane.
The use of commercially available planning software allows planning of target and trajectory. Planned trajectories that avoid the cerebral sulci and ventricles would avoid the intrasulcal and intraventricular vessels, theoretically minimizing the risk of hemorrhage.
Direct Localization
STN
The STN is a popular and effective target in the symptomatic management of PD. Models of information flow through the basal ganglia suggest that this nucleus is overactive in PD with abnormal synchronization of neuronal activity in the beta range (13–30 Hz). Lesioning or chronic high-frequency stimulation of this nucleus is believed to disrupt abnormal neuronal activity and restore some balance to the corticobasal ganglia circuitry involved in control of movement. Tens of thousands of patients have undergone STN DBS with, on the whole, beneficial effects on bradykinesia, rigidity, tremor, and motor fluctuations.
Located in the rostral mesencephalon, the STN is a small biconvex structure (3 × 5 × 12 mm) lying posteromedial to the crus cerebri; its longitudinal axis is obliquely oriented such that the superior pole lies lateral and posterior to the inferior one. Functional segregation of the nucleus into motor, cognitive, and limbic portions mirrors that of other basal ganglia structures. Extrapolation from animal studies and MRI localization of the most effective active DBS contacts suggest that the motor component of the STN is located in its most superior portion.
The STN can be visualized on MRI, allowing direct targeting of this structure ( Fig. 4 ). On T2-weighted images, the STN presents a characteristic hypointense signal that has been attributed to the presence of iron. Bejjani and colleagues proposed the red nuclei as an internal landmark to the STN (see Fig. 4 ). Concerns have been raised about discrepancies between the expected size of the STN on MRI and that seen on histologic atlases. This may represent a relative paucity of iron deposits within the posterior portion of the nucleus, thus rendering part of the nucleus less visible on MRI. Nonetheless, stereotactic localization is better served by direct visualization of part of the target structure than by estimating its location based on stereotactic atlases.
Globus pallidus internus
Forming the most medial part of the lentiform nucleus, the globus pallidus internus (GPi) lies medial to the lamina interna and lateral to the internal capsule. Numerous investigators targeted the ansa lenticularis and pallidum in the early decades of the twentieth century, with Leksell noting superior results in the posteroventral region. Motor function was later mapped to the posteroventral portion of the GPi in animal studies, confirming Leksell’s clinical observations, and this is now the accepted surgical target in the treatment of various dystonias and dyskinesia.
MRI protocols that allow clear visualization of the pallidal architecture have been published. The author uses a modified proton-density sequence for targeting the posteroventral pallidum ( Fig. 5 ). The putamen, internal and external pallidum, and the pallidocapsular border can easily be seen at the level of the AC. This sequence guides the surgical trajectory to the posteroventral pallidum as it lies immediately superior and lateral to the optic tract.
Motor thalamus and zona incerta
The motor thalamus (Vim, Vop and Voa/VLp: Hassler/Hirai and Jones classifications respectively) is an excellent surgical target for addressing tremor. Visualization of individual thalamic nuclei is unreliable at 1.5 T although attempts have been made to visualize these at higher field strengths. Indirect targeting currently retains an important role in the use of this target, although the lateral and anteroposterior coordinates can be guided by visualization of the thalamocapsular border on CT or MRI.
The zona incerta (ZI) is continuous with the inferior aspect of the thalamic reticular nucleus, and has been proposed as an alternative target to motor thalamus and STN for tremor and parkinsonism respectively. Lying on the superomedial aspect of the STN, and medial to its most superior and lateral tail, direct ZI localization is possible on T2-weighted scans ( Fig. 6 ). It is usually possible to plan a trajectory that will allow a quadripolar DBS electrode to straddle ZI and motor thalamic targets.
Pedunculopontine nucleus
The pedunculopontine nucleus (PPN) forms part of the rostral locomotor region of the brainstem and is believed to play a central role in the initiation and maintenance of gait. An elongated neuronal collection in the lateral pontine and mesencephalic tegmental reticular zones, the nucleus straddles the pontomesencephalic junction, its long axis roughly parallel to that of the fourth ventricle floor. The rostral pole lies at midinferior collicular level, the nucleus extending circa 5 mm caudally to reach the rostral pons. A triad of projection pathways circumscribes the region of the PPN: the superior cerebellar peduncle and its decussation, the central tegmental tract, and the lemniscal system ( Fig. 7 ). Translational research has led to promising reports of DBS in the rostral brainstem in humans. However, this region of the brain is unfamiliar territory to most functional neurosurgeons, and has been the subject of inaccurate descriptions and representations. Images acquired using a specifically modified proton-density MRI protocol provide excellent definition between gray and white matter within the region of interest at 1.5 T. This method, together with an understanding of the regional anatomy, allows accurate PPN localization on stereotactic MRI in clinical practice (see Fig. 7 ).
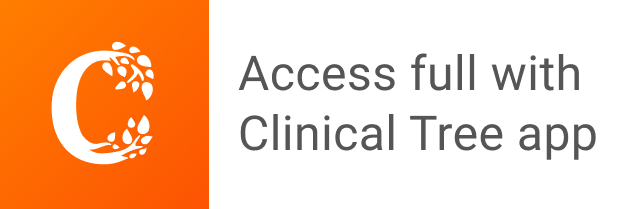