Fig. 5.1
Minimal fracture line (white arrow) visible in the ventral cortex of the tibia on conventional radiography of a 26-year-old female
The chronological findings on magnetic resonance imaging (MRI), as golden standard in the diagnosis of stress injuries, are oedema followed by a cortical fracture line (Fredericson et al. 2006). Pathophysiologically, repetitive stress causes disbalance in the bone remodelling process with microfractures, extensive oedema and haemorrhage. This oedema, either periosteal or in the bone marrow, is represented by increased signal intensity on STIR or fat-saturated T2-weighted MR images (Fredericson et al. 2006; Gaeta et al. 2005) (Fig. 5.2).
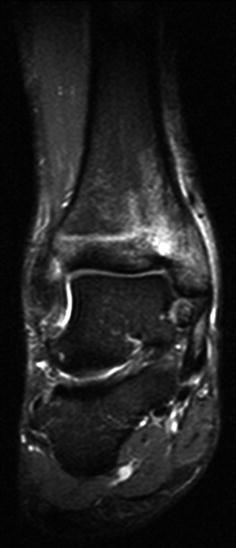
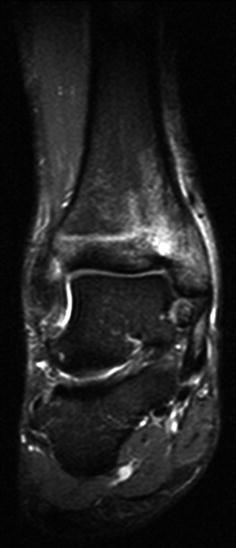
Fig. 5.2
Medial tibia stress fracture in a 19-year-old professional tennis player, indicated by high signal intensity on a coronal T1 TIRM sequence
Ultrasound can also be helpful in the detection of stress injuries, by showing cortical defects and a hyporeflective layer of oedema, indicative of non-ossified periosteal reaction (Allen and Wilson 2007). The sensitivity and specificity of ultrasound were 81.9 and 66.6 %, respectively, in comparison with MRI in the detection of stress injuries in the lower limb of athletes (Papalada et al. 2012).
In the clinical setting, MRI is frequently followed by computed tomography (CT) to tailor the individual approach in radiologic-orthopaedic multidisciplinary setting (Fig. 5.3). The major advantage of CT over MRI is the direct visualisation of calcified periosteum and bony structures to show the possible cortical fracture line. CT is also valuable for stress injuries in complex anatomical sites, for longitudinal stress fractures and in the differential diagnosis with tumoral lesions, for instance, osteoid osteoma (Demeijere and Vanhoenacker 2007).
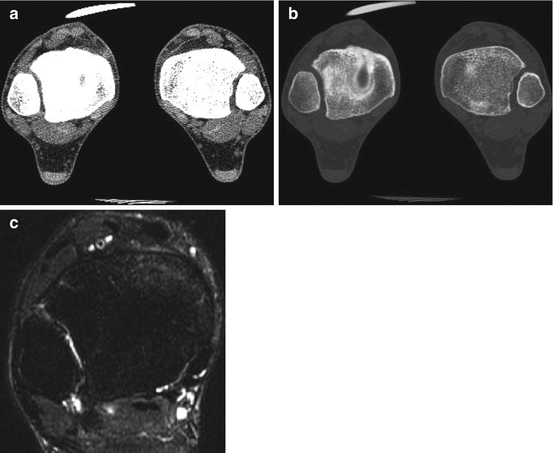
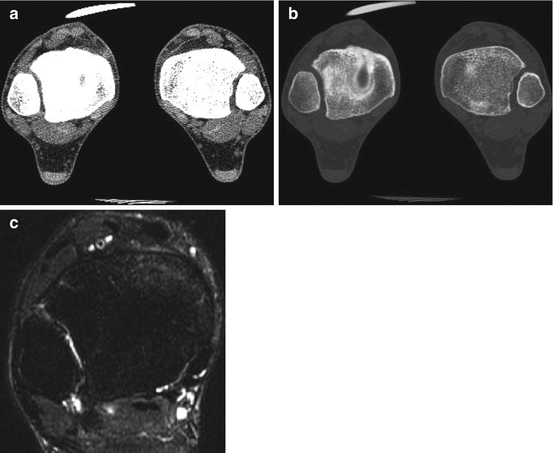
Fig. 5.3
CT of the ankle shows subtle abnormalities belonging to bone contusion at the distal tibia in an 18-year-old female gymnast. The importance of adequate windowing is emphasised by the difference between (a) soft-tissue window and (b) bone window setting. An axial T2-weighted MR image (c) with fat saturation at the same level shows very subtle high signal intensity, indicative of bone marrow oedema. This abnormality might not be obvious enough for the diagnosis of a bone bruise, thereby emphasising the need for additional CT in this case
Despite the specific (dis)advantages of each imaging technique, they share a potential additional value in the diagnostic and follow-up process of stress reactions. To assort the heterogeneous presentation of the stress-related bone disease and its imaging presentation, a general modality- and site-independent grading system for stress fractures has recently been developed (Kaeding and Miller 2013).
5.2.2 Imaging in the Follow-Up of Stress Injuries
Imaging can predict the course of stress injuries in their follow-up period. Besides the location of the stress injury, prognostic factors like MRI grade at diagnosis can be very valuable in predicting the time to full return (Dobrindt et al. 2012; Nattiv et al. 2013). The duration of the rest period after the diagnosis of stress injury depends on the location, the patient’s individual characteristics and the type of sport.
Repeated imaging is not an absolute requirement before return to activity. If plain radiography is performed, no more abnormalities besides callus should be present (Royer et al. 2012). When MRI is repeated, one should carefully interpret the findings and always correlate with clinical symptoms, because firstly the presence of features mimicking stress injuries is present in approximately 43 % of asymptomatic athletes (Bergman et al. 2004). Other studies state that MRI features of stress injuries resolve at longer term and are also hampered by susceptibility artefacts of the thick sclerotic cortical bone next to the original fracture line. CT is believed to be more accurate in the long-term detection of stress fractures than MRI (Fig. 5.4) (Burne et al. 2005).
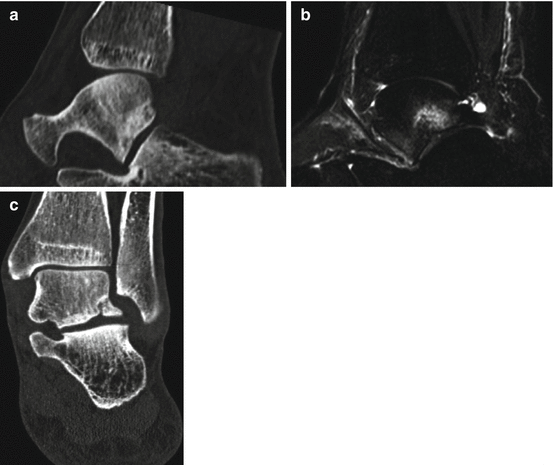
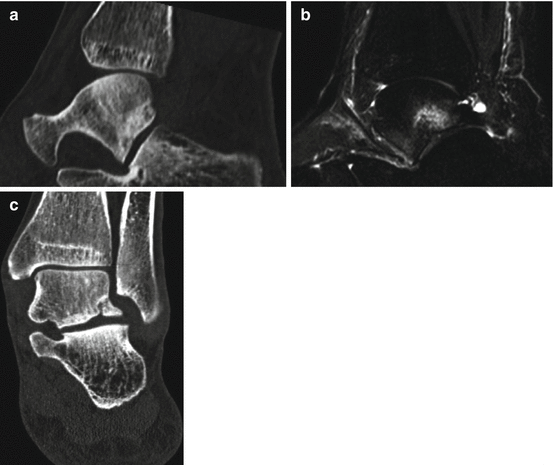
Fig. 5.4
Stress fracture of the lateral process of the talus on CT of the ankle in a 60-year-old male runner visualised on CT (a) and MRI (b). The CT shows sclerosis in the talus with a subtle fracture line, which may be hard to detect. On a sagittal T2-weighted MR image with fat saturation, an evident area of high signal intensity is present, clearly indicating the stress fracture. Follow-up CT (c) performed 17 months later (c) shows no complete healing of the abnormalities in the coronal orientation. Bone resorption in the original fracture line is visible
5.3 Cartilage Injuries
Cartilage is a frequently affected tissue in athletes. Spontaneous healing of cartilage injuries is extremely rare and persistent damage can lead to osteoarthritis. Imaging is a key player in the management of cartilage injuries in athletes, as adequate depiction of abnormalities enables good decision-making both in the diagnostic and follow-up phase.
Currently the imaging of cartilage injuries in sports medicine is based on MRI and MR arthrography as golden standards. The MRI field strength of 3.0 T is superior to 1.5 T in both morphologic and compositional imaging of the cartilage. The use of 7.0 T in cartilage imaging is still restricted to research purposes (Roemer et al. 2011). Although MRI is used more often in daily practice for cartilage injuries, there is no significant difference of diagnostic accuracy between MRI and CT in, for example, the detection of osteochondral defects (Verhagen et al. 2005). Plain radiography can be helpful for, for example, assessing weight-bearing joint space width. Arthrography without subsequent CT or MRI is no longer performed (Huysse and Verstraete 2007). The comparable diagnostic accuracy of CT arthrography to MR arthrography is overruled by drawbacks like radiation dose and inability to visualise bone marrow oedema (Waldt et al. 2005). CT arthrography is indicated when patients cannot undergo MRI due to contraindications (De Filippo et al. 2009).
5.3.1 Cartilage-Specific MRI Sequences
5.3.1.1 Morphologic Assessment of Cartilage
There is a large group of sequences available for morphologic assessment of cartilage, roughly to be divided in (fast) spin echo (FSE) and gradient-recalled echo (GRE) sequences. Besides the more conventional SE sequences, recently many new MRI techniques with high accuracy for depicting cartilage have been developed. The vast majority of the cartilage-specific MRI sequences perform similarly with respect to diagnostic accuracy. Other characteristics such as acquisition time, utility for other structures and susceptibility to artefacts are distinctive and summarised in Table 5.1.
Table 5.1
MRI techniques for morphologic evaluation of cartilage
Technique | Diagnostic accuracy for cartilage lesionsa | Other structures | Acquisition time | Signal intensity | ||
---|---|---|---|---|---|---|
Cartilage | Fluid | |||||
(F)SE | 2D FSE (T2 or PD) | Sens 68.2 % | + | – | Intermediate | High |
Spec 92.8 % (Kijowski et al. 2009a) | ||||||
3D FSE | Sens 72.8 % | + | + | Intermediate | High | |
Spec 88.2 % (Kijowski et al. Kijowski et al. 2009b) | ||||||
SPACE | Sens 82.3 % | Unknown | – | Intermediate | High | |
Spec 80.2 % (Notohamiprodjo et al. 2012) | ||||||
3D DEFT (DRIVE) | Sens 100 % | Unknown | – | Intermediate | High | |
Spec 58.3 % (Yoshioka et al. 2004) | ||||||
GRE | 3D SPGR | Sens 96.7 % | – | – | Very high | Low |
Spec 85.3 % (Yoshioka et al. 2004) | ||||||
FLASH | Sens 62.4–74.2 % | Unknown | – | Very high | Low | |
Spec 77.6–89.4 % (Duc et al. 2007) | ||||||
3D DESS | Sens 55.9–72.0 % | Unknown | + | Intermediate | Very high | |
Spec 95.3–77.6 % (Duc et al. 2007) | ||||||
3D bSSFP (trueFISP) | Sens 51.6–64.5 % | + | + | High | Very high | |
Spec 80.0–94.1 % (Duc et al. 2007) | ||||||
VIPR | Sens 76.5 % | + | + | Intermediate | Very high | |
Optimal visualisation of cartilage with MRI encompasses several challenges: adequate depiction of accompanying bone marrow oedema, avoiding MRI artefacts, short acquisition time, etc. Some disadvantages can be addressed to a group of sequences. For example, the fat suppression and concomitant adequate depiction of bone marrow abnormalities is particularly achieved with non-GRE-type sequences (Roemer et al. 2009). Additionally, GRE-type sequences are also more prone to susceptibility artefacts caused by, for example, metal. Furthermore two-dimensional sequences are hampered by obligatory acquisition in multiple planes, which increases the total scanning time. On the other hand, the longer duration of three-dimensional sequences goes together with higher susceptibility to motion artefacts.
In clinical practice, the standard sequence mostly used for the evaluation of cartilage is a two-dimensional (2D) fast spin echo (FSE) sequence, preferable T2- or PD-weighted sequences. T1-weighted FSE images are avoided due to poor capability of depicting other structures besides cartilage and lack of contrast between joint effusion and cartilage. The development of three-dimensional (3D) FSE sequences with multiplanar reconstruction capabilities improves acquisition time and is very promising for future clinical use (Roemer et al. 2011).
5.3.1.2 Compositional Imaging of Cartilage
Very early damage to the articular cartilage cannot be reliably detected by any of the MRI sequences from Table 5.1 (Huysse and Verstraete 2007). Novel MRI imaging techniques depicting the changes on biomechanical or structural level can be of great additional value in the detection of this early cartilage degeneration in athletes. The most important compositional imaging techniques include T2 mapping, delayed gadolinium-enhanced MR imaging of cartilage (dGEMRIC), T1ρ imaging, sodium imaging and diffusion-weighted imaging. They are all based on assessment of collagen network, water content and/or glycosaminoglycans (Crema et al. 2011). However, these quantitative MRI techniques need further refinement before any additional value in cartilage imaging in sports can be guaranteed.
5.3.1.3 MR Arthrography
MR arthrography can be performed in a direct and indirect manner. The former includes direct intra-articular injection of contrast solution, mostly under fluoroscopic visualisation. Indirect MR arthrography is the intravenous contrast administration followed by MRI. In cartilage imaging, direct MR arthrography is useful in smaller joints and joints with thinner cartilage surfaces. The most frequently imaged joints by direct MR arthrography in the athletic population are the hip and the shoulder joint. Diagnostic accuracy for the detection of cartilage lesions for both the hip and shoulder joint is dependent on the level of training of the performing radiologist (McGuire et al. 2012; Theodoropoulos et al. 2010). Nevertheless, sensitivity and specificity of direct MR arthrography for the detection of cartilage injuries in the hip and shoulder are mostly reported to be superior to conventional MRI (Blankenbaker and De Smet 2010; Lee et al. 2013; Magee 2009).
5.3.2 Cartilage Abnormalities on MRI
The underlying pathophysiologic mechanisms of the cartilage defects on MRI can roughly be divided into acute/traumatic and chronic for every joint. An MRI abnormality indicative of acute cartilage injury is subchondral bone marrow oedema. Bone marrow oedema accompanying a chondral defect reflects the response of the subjacent bone to acute injury, following impact or shear forces on the cartilage. In case of subcortical broad-based bone marrow abnormalities on MRI, an osteochondral fracture needs to be ruled out. An osteochondral fracture is characterised by involvement of both the cartilage and the subchondral bone, visualised as an interruption of the subchondral ‘black line’ on MRI (Black et al. 2009). Bone marrow oedema can also be present in a focal cartilage lesion (also referred to as ‘transchondral fracture’), in which there exists only a partial- or full-thickness interruption of the cartilage on MRI (Black et al. 2009). Furthermore osteochondritis dissecans and chondral delamination have an acute injury mechanism as well. Abnormalities as sclerosis of the subchondral bone, gradual lesion edges and absence of bone marrow oedema shift probability more towards a chronic cartilage lesion (Huysse and Verstraete 2007) (Fig. 5.5).
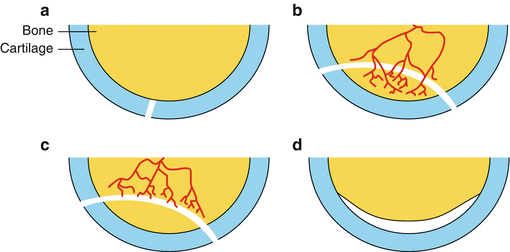
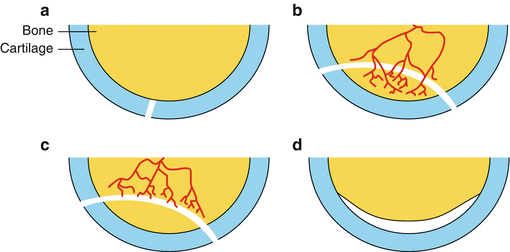
Fig. 5.5
(a–d) Schematic representation of injuries involving cartilage: (a) focal cartilage lesion, (b) osteochondral fracture, (c) osteochondritis dissecans and (d) cartilage delamination
5.3.3 Radiologic Follow-Up of Cartilage Injuries
As cartilage has very limited intrinsic repairing capabilities, surgical repair is often warranted to prevent osteoarthritis at long term. For the evaluation of this cartilage repair on MRI, several classification systems have been developed. The magnetic resonance observation of cartilage repair tissue (MOCART) score is reliable, reproducible and accurate, and therefore most frequently used (Marlovits et al. 2004; Marlovits et al. 2006). Graft failure is more likely when MRI signs like persistent marrow oedema (>8–12 months), subchondral cyst formation, extensive subchondral sclerosis, narrow thickness of graft and delamination of the cartilage are present. Despite the reliability as a cartilage repair MRI evaluation tool, the capability of the MOCART score to predict clinical outcome is questionable (de Windt et al. 2013).
5.4 Tendon and Ligament Injuries
Tendons and ligaments share their longitudinal orientation of dense connective tissue. However, ligaments have more interweaving of collagen fibres in comparison with tendons.
Sports-related tendon injuries can be divided in tendon tears, tendinopathy (the former tendinosis), tendon subluxation and tenosynovitis. The term ‘tendinitis’ is confusing, because it implies inflammation of the tendon but refers to the same noninflammatory degenerative condition as tendinosis (Maffulli et al. 1998). Tendinopathy is a more descriptive umbrella term comprising the clinical syndrome (Chang and Miller 2009).
Recently, a pathology model has been developed to explain the tendinopathy presentation (Cook and Purdam 2009), in which tendinopathy is suggested to be a continuum, ranging from reactive tendinopathy to tendon disrepair and ultimately to degenerative tendinopathy. Tendon ruptures are more likely to occur at the weak point in the kinetic chain suffering from, for example, repetitive overuse (Kainberger and Weidekamm 2005), i.e. the so-called degenerative tendinopathy stage (Cook and Purdam 2009). For the performing radiologist, it is important to look for unique and distinguishing features of each individual tendon abnormality (Fig. 5.6).
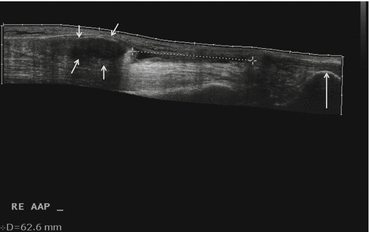
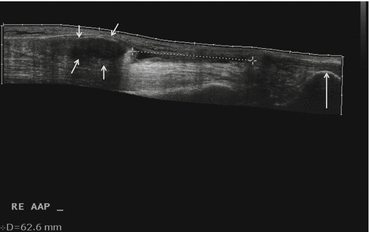
Fig. 5.6
Sagittal image in 56 year old male patient with sudden painful snap at the right calf during running activity. The patient has a history of chronic activity (several months) related pain at the right calf. Sagittal US extended-field-of-view-scan shows retracted tendon with empty paratenon (cross marks). Proximal retracted and thickened tendon (short arrows), distal tendon insertion at tuber calcanei (long arrow) has a normal appearance (Image provided by JLMA Gielen, University of Antwerp)
Ligament injuries of an athlete include both acute and chronic conditions but can also be classified as intra- and extra-articular injuries. Typical examples of sports-related tendon and ligament injuries include the rotator cuff and Achilles tendinopathies, jumper’s and runner’s knee, tennis and golfer’s elbow, climber’s finger and ankle sprains.
5.4.1 Imaging Tendon Injuries
The majority of tendon and ligament abnormalities are detected in soft-tissue structures, limiting the utility of plain radiography or CT in these injuries. It is stated that CT is only useful in case of bone trauma, for the detection and assessment of subtle fractures. However, in our experience CT is also necessary to rule out tiny bone fragments accompanying the tendon injury. These bone fragments are not easily detected with MRI or US but can cause chronic irritation and suboptimal recovery.
Both ligaments and tendons are susceptible to anisotropy on ultrasound when the ultrasound beam is not projected perpendicular to the fibres of the tendon or ligament. This phenomenon is visible on ultrasound as loss of echogenic appearance and may simulate disease of the tendon or ligament (Garcia et al. 2003). Although ultrasound is easy to use and allows dynamic evaluation, MRI has certain advantages in imaging tendon disease as well. Besides the ability to evaluate deeper located structures, abnormalities in surrounding tissue accompanying the tendon disease (e.g. bone marrow oedema) can also be detected (Kainberger and Weidekamm 2005). However, also on MRI an anisotropic prolongation of relaxation time can occur on short echo sequences when the tendon is oriented approximately 55° to the magnetic field. This phenomenon is called the magic angle phenomenon and mimics tendinopathy because of the artificial high signal intensity in the tendon (Erickson et al. 1991). Both modalities show high accuracy in the detection of various tendon abnormalities (Chang and Miller 2009).
5.4.1.1 Tendon Abnormalities on US
A full-thickness tendon tear can also be accompanied by a haematoma and needs to be differentiated from partial-thickness tear or tendinopathy, due to the need for surgical intervention (Tok et al. 2012). Dynamic US can be very helpful in showing a gap in the tendon in case of a tear and to dynamically assess tendon subluxation (Allen and Wilson 2007; Neustadter et al. 2004).
Tendinopathy is visualised on ultrasound by features as tendon thickening, heterogeneous echotexture, focal hypoechogenicity and calcifications (Ahmed and Nazarian 2010). An abnormality which tendinopathy has in common with tenosynovitis is increased power Doppler flow in the tendon. In case of tenosynovitis, this neovascularisation depicted as increased Doppler flow can also be detected in the tendon sheath. Though the feature on ultrasound is the same, the underlying pathophysiologic mechanism of the neovascularisation is different: inflammation in tenosynovitis and attempt of repair in tendinopathy (Allen 2007).
5.4.1.2 Tendon Abnormalities on MRI
The most common MRI abnormalities that can be present in every pathophysiologic phase of sports-related tendon disease include increased intratendinous signal intensity and thickening of the tendon. Tendon sheaths or paratenons which enhance after intravenous contrast or surrounded by fluid are more specific for tenosynovitis. In case of partial- or full-thickness tendon tear, the radiologist should look for discontinuity of the fibres and a tendon gap, filled with either fluid, fat or scar tissue (Chang and Miller 2009; Hodgson et al. 2012).
5.4.2 Imaging Ligament Injuries
The modality of choice for ligament injuries depends on the suspected anatomic location and injury mechanism: intra-articular ligament injuries are exclusively properly visualised with MRI, while extra-articular ligaments can also be visualised by US (Allen 2007). Although MRI may be technically more difficult (e.g. small section thickness needs to be obtained), the capability to assess other abnormalities (e.g. microfracturing) is advantageous.
The radiologist needs to be aware of the injury mechanism, as acute and chronic ligament sprains require other imaging techniques and represent with other imaging characteristics. An acute ligament sprain often shows fluid around the ligament, presenting as hypoechoic on US and with increased signal intensity on STIR or T2-weighted MR images. Moreover the original fibre structure of the ligament is disturbed. In case of an acute injury mechanism possibly leading to ligament rupture, imaging is the key player in distinguishing partial from complete ruptures. The latter are characterised by discontinuity of the ligament, a haematoma and instability or gaps at dynamic US examination. Chronically damaged ligaments show laxity and irregularities on imaging. Also residual features like calcifications or even nonunion can be visualised both on US and MRI (Allen 2007; Hodgson et al. 2012).
5.5 Muscle Injuries
The muscle is affected in approximately one third of all injuries in athletes (Junge and Dvorak 2013). Although the clinical examination may sometimes be sufficient, imaging is very important for the diagnosis, prognosis and monitoring of muscle injuries in athletes. Muscle lesions in athletes are mostly divided based on injury mechanism: direct, indirect or late complications of muscle injuries, as visualised in Fig. 5.7 (Mueller-Wohlfahrt et al. 2013). Also imaging-based classification systems have been developed for both MRI (Ahmad et al. 2013) and US (Peetrons 2002). MRI is considered as the golden standard technique, as it is more sensitive in follow-up imaging (Connell et al. 2004). Nevertheless US also has some interesting advantages in the evaluation of muscle injuries, further specified in Sect. 5.5.2.1. Additionally, the development of novel imaging techniques, like diffusion-tensor imaging (DTI) and US-related techniques (e.g. elastography), provides new perspectives in the research field of muscle injuries.