Introduction
Nearly 2 million cases of traumatic brain injury (TBI) occur each year. Worldwide, trauma is the leading cause of death and disability in those younger than 45 years, with nearly half of fatalities resulting from TBI. In younger patients, traffic accidents are the leading cause of TBI, and in the elderly, falls are the principal cause. TBI is a heterogeneous disorder with different forms of presentation. Approximately 15% to 25% of TBIs are serious injuries requiring specialist care, but the majority are considered mild. Mortality from severe TBI has decreased substantially with protocols that depend in part on computed tomography (CT) to monitor and recognize findings that may result in further injury. Magnetic resonance imaging (MRI) is an important problem-solving tool in moderate to severe TBI and has shown increasing utility for risk-stratifying mild TBI (mTBI), which can be associated with insidious long-term cognitive impairment.
Classification of Traumatic Brain Injury
TBI is most broadly classified based on blunt or penetrating mechanism. This chapter emphasizes issues pertaining to the imaging of TBI from blunt head trauma. TBI may be intra- or extraparenchymal ; intraaxial injuries are further subdivided as focal or diffuse, the former occurring more commonly from falls in the elderly and the latter in high-velocity traffic accidents. Injury may be primary, resulting directly from external forces and structural strain to cerebral tissue at the time of the traumatic event, or secondary, representing evolution of the primary injury as well as consequences and complications of increased intracranial pressure (ICP).
Mechanism of Injury—Primary TBI
Intraaxial Lesions
Diffuse Axonal Injury, Contusions, and Intracerebral Hematomas.
TBIs usually results from either deceleration impact or impulsive acceleration and deceleration forces (e.g., “whiplash”), which cause variable degrees of compression, tension, and shear. Shear stress is caused by differential acceleration between peripheral and central portions of the brain with respect to the axis of rotation (often about the fulcrum of the cervical spine or from wave propagation through the head after direct impact. Diffuse axonal injury (DAI) is seen in approximately half of severe TBI cases and refers to the microstructural damage to white matter tracts resulting from shear injury. Injury occurs primarily at interfaces of different density such as the gray-white matter junction and the corpus callosum, and also tends to cluster where the brain can collide and splay out against dural reflections such as the falx and tentorium. This may account for the predilection of DAI to the midline of the splenium and the dorsolateral midbrain ( Fig. 13-1 ). Generally the more central the injury, the greater the overall severity, and severity is greatest when the midbrain or brainstem is involved. Contusions occur in approximately 45% of patients with TBI. Coup contusions are relatively common in falls that result in lateral and occipital blows to the calvarium. These are sometimes seen deep to the margins of depressed skull fractures ( Fig. 13-2 ). Because coup contusions tend to involve the cortex immediately subjacent to the point of impact, as in DAI, contusions extending more centrally also tend to be more severe. Stress waves resulting from motion of the buoyant brain against the fixed inelastic calvarium and skull base account for the frequent presence of both coup and contrecoup contusions. Contrecoup contusions are usually more severe and are also more common than coup contusions, possibly owing to cavitation effects as the brain reflects off the inner table of the skull and the gliding movement of the undersurface of the brain over the rough surface of the anterior and middle cranial fossae. This also explains the susceptibility of the orbitofrontal regions (especially the gyrus rectus) and anterior inferior temporal lobes to contrecoup contusions ( Fig. 13-3 ). Intraparenchymal hematomas involving deeper structures such as the thalamus, basal ganglia, hippocampus, and parahippocampal regions are distinct from cortical contusions, and like DAI can result from shear strain or may be caused by compression against the tentorium after blows to the vertex.
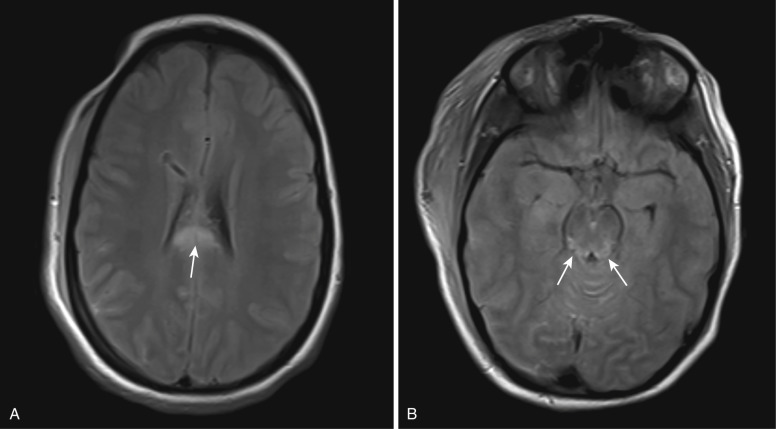
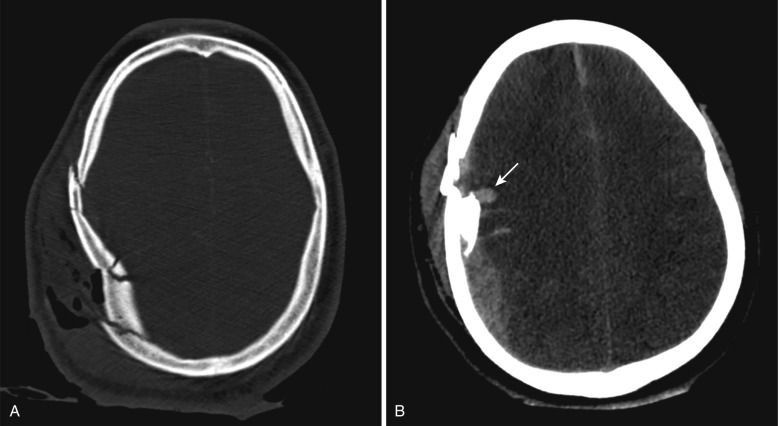
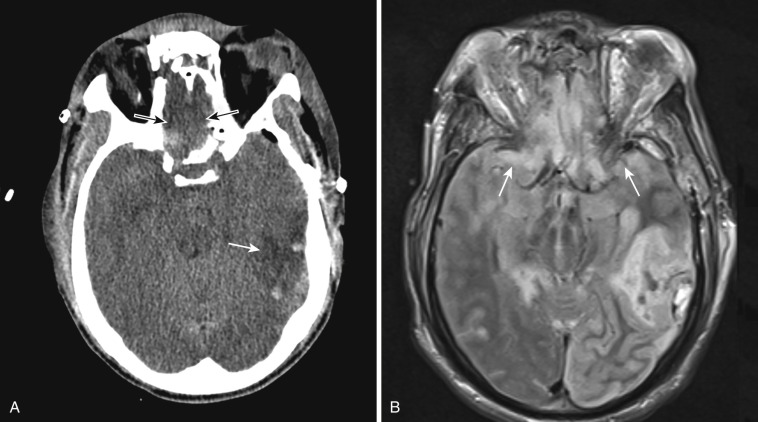
Extraaxial Lesions
Tensile failure of bridging veins that extend from the subarachnoid perivascular spaces to the dura accounts for the high frequency of subdural hematomas (SDH), particularly in the elderly and in children, where the vessels are maximally preloaded as a result of increased extraaxial space. The subdural space is normally occupied by fluid similar to cerebrospinal fluid (CSF). Because of this, the greatest amount of motion occurs in the subdural space, predisposing bridging veins to shearing. SDHs tend to spread out along the entire hemisphere because there is very little attachment between the inner leaflet of the dura and the arachnoid ( Fig. 13-4 ). SDHs sometimes occur as a result of isolated impulsive forces without impact for this reason. Tearing of cortical veins resulting in SDH often occurs in the same distribution as coup and contrecoup contusions, and like contusions, a large proportion of SDHs, epidural hematomas (EDHs), and subarachnoid hemorrhage (SAH) result from lateral blows.
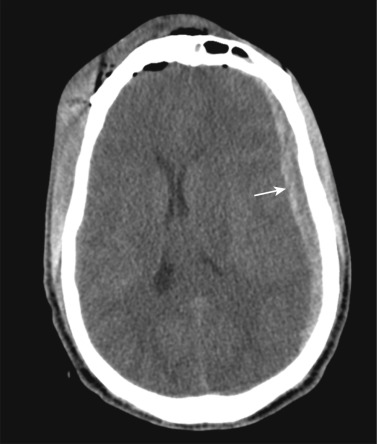
Knowledge of the anatomy of fluid spaces and the relationship of the meningeal layers to the brain surface and inner table of the skull is important to understand common appearances of different extraaxial hemorrhagic lesions. The pia lines the cortical gyri as well as perforating vessels (bridging veins), forming a perivascular space (Virchow-Robin space) that communicates with the subarachnoid space, lying between the arachnoid and pia. SAH can result from laceration of small cortical, pial, or subarachnoid vessels in the subarachnoid space, or the pial/subarachnoid portions of bridging veins. SAH may also result from transependymal diffusion of intraventricular hemorrhage (IVH) if the ependyma lining the ventricles is compromised, from direct migration through the ventricular outflow foramina, from direct extension of hemorrhage within a cortical contusion, from SDH with arachnoid tear, and from EDHs with dural and arachnoid tears.
EDHs are more closely correlate with skull fractures than SDH and often occur in the temporoparietal region because the middle meningeal artery, which runs within a groove in the inner table of the squamous temporal bone, or its branches are the usual source of bleeding. The dura is a two-layer membrane with an inner meningeal layer and outer periosteal layer that is tightly bound to the skull. The two layers encase the venous sinuses. The epidural space is a potential space between the periosteal layer of the dura and inner table of the skull. The periosteal layer of the dura is tightly adherent to the inner table, particularly at the sutures, preventing most EDHs from crossing sutural margins ( Fig. 13-5 ). Small EDHs without clinical deterioration can be treated conservatively but need to be followed closely because approximately one quarter enlarge within 36 hours after injury, usually within the first 5 to 8 hours ( Fig. 13-6 ). IVH may result from shearing of subependymal veins during rotational acceleration, direct extension from parenchymal hematoma, or from retrograde migration through ventricular outflow foramina.
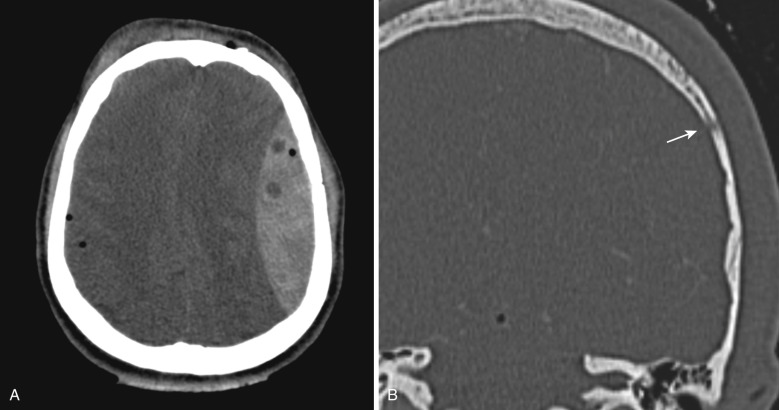
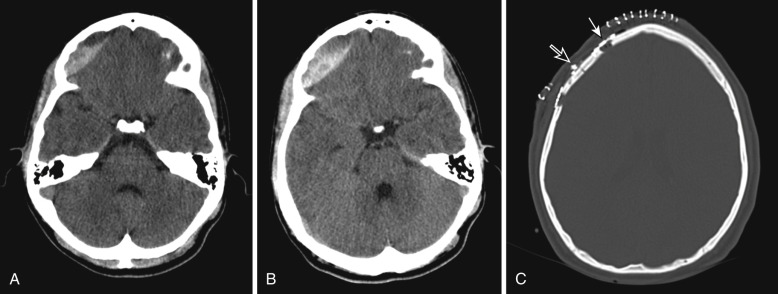
Mechanism of Injury—Secondary TBI
TBI is not a one-time event but rather a continuously progressive injury. Secondary brain injury may refer to the evolution and enlargement of the primary injury around a “traumatic penumbra” (analogous to the ischemic penumbra in stroke) ( Fig. 13-7 ), or it may refer to the potentially preventable consequences of resultant mass effect and elevated ICP, including herniation, ischemia and infarction, and global hypoxic injury. Secondary brain injury is responsible for over 50% of deaths related to trauma within the first 48 hours after injury. Brain swelling after TBI can be the result of both cytotoxicity and vasogenic edema, which have a synergistic effect. The initial mechanical disruption results in cellular damage and cytotoxicity that progresses throughout the first week after injury. Ischemic damage after primary brain injury is most commonly perilesional, resulting from impaired cerebral perfusion and oxygenation, excitotoxic injury, focal arterial or venous microthrombi, and vasospasm. On a cellular level, traumatic injury induces a vicious cycle beginning with the release of excitatory neurotransmitters such as glutamate, which causes a massive influx of calcium and sodium into neurons, leading to cellular swelling, mitochondrial dysfunction, free radical production, and calcium-mediated proteolysis. This in turn leads to ischemic and apoptotic cell death and more neurotransmitter release. Although cytotoxic edema appears to be the primary cause of cerebral swelling after TBI ( Fig. 13-8 ), the blood-brain barrier typically becomes compromised, leading to some degree of vasogenic edema. Mass lesions and swelling cause increased ICP, with resulting herniation that can occlude vessels and cause territorial infarction ( Fig. 13-9 ). During the subacute period after the initial injury, microglial cells enter areas of focal injury and phagocytize blood products and damaged tissue. A cavitary area of encephalomalacia lined by glia results ( Fig. 13-10 ). Small lesions may heal only with a glial scar. The overall effect is widespread volume loss beyond any focal area of TBI.
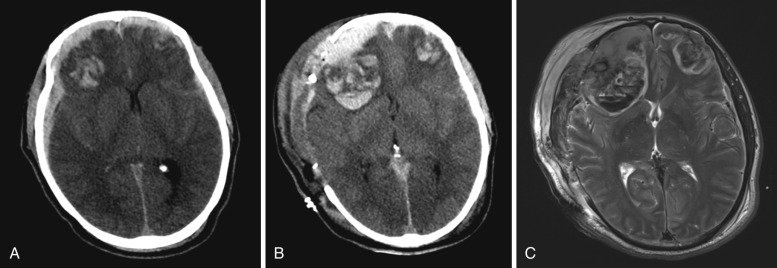
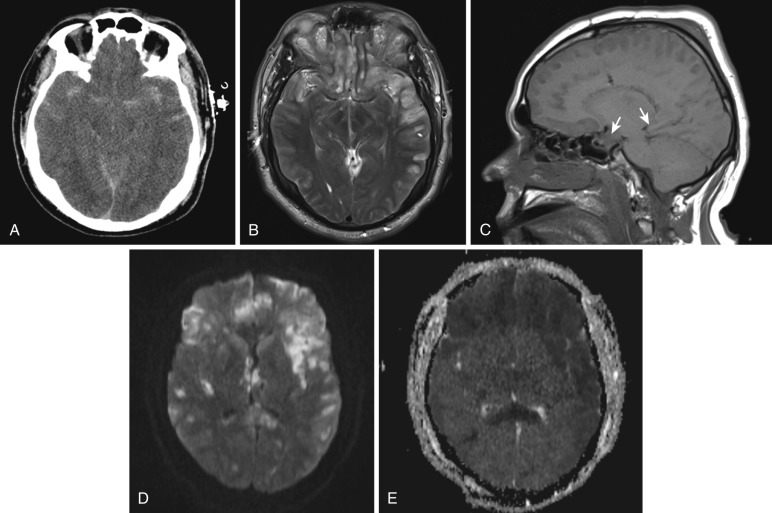
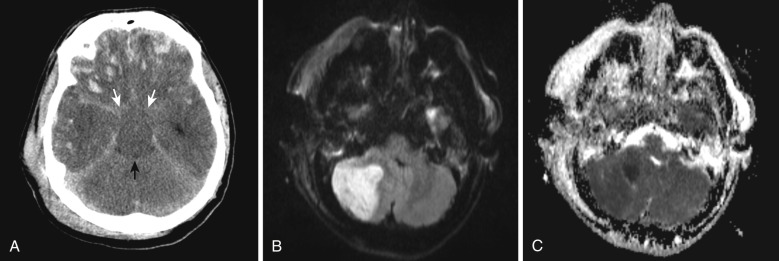
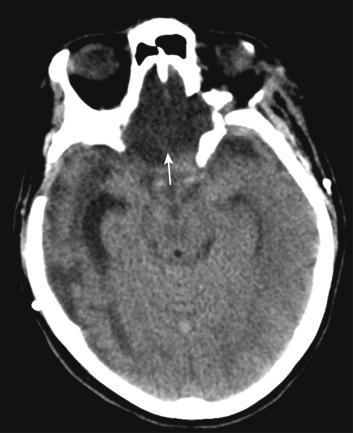
Indications for Imaging
The Glasgow Coma Scale (GCS) is a ubiquitously used scoring system for clinical grading of TBI severity, with eye, verbal, and motor components added to give a sum score ranging from 3 to 15. The lowest score in the first 48 hours is used to classify TBI. Scores of 13 to 15 correspond with mild TBI, scores of 9 to 12 with moderate TBI, and scores of 3 to 8 with severe TBI. In practice, the GCS can falsely suggest brain injury in the setting of intoxication, sedation, or paralysis. Another limitation is that most patients with TBI have mild injuries and may exhibit residual neurocognitive sequelae despite having normal or near-normal GCS scores. Nevertheless, the GCS serves as a useful triage tool for determining the need for CT. CT is generally indicated for severe and moderate TBI (GCS < 13). Some argue for imaging any patient with a GCS below 15, even though less than 20% of patients with minor head trauma have positive findings on CT and only up to 5% require intervention. Additional factors, such as high-velocity mechanism, contribute to the likelihood of positive CT findings even when GCS scores are normal. Other predictors used to determine the need for head CT in otherwise clinically mild head injury include risk factors such as advanced age (>60-65 years), loss of consciousness greater than 5 minutes, worsening level of consciousness, failure to improve over time, periods of transient amnesia or confusion, and headache with episodes of vomiting. Scanning should be performed more liberally in those on anticoagulation therapy or with bleeding diatheses and should be routinely performed for depressed skull fractures. CT is also important in allowing the neurosurgeon to clear patients prior to surgical treatment of non–life-threatening extracranial injuries. In unconscious patients, fixed dilated pupils are a sign of herniation requiring urgent imaging and decompression. Deteriorating mental status and delayed development of coma may reflect evolving secondary injury with worsening ICP and herniation or brainstem compression. DAI, which may not be apparent on CT, can also be the sole source of posttraumatic coma.
Because of the time, resources, and logistics involved in MR scanning, particularly in polytraumatized patients with anesthetic and monitoring equipment, CT has long been used as the workhorse to screen patients for prognostic indicators for surgical intervention and for following the dynamics of lesion development, although MRI remains an important problem-solving tool. Any focal lesions detected on CT, regardless of size, require follow-up CT scanning. New lesions will arise in around 15% of patients, and 25% to 45% of cerebral contusions will significantly enlarge (see Fig. 13-7 ). CT progression generally occurs within 6 to 9 hours after injury. CT facilitates a preemptive approach in which surgery is used to prevent deterioration rather than treating lesions once neurologic deterioration has already occurred. This strategy has been shown to improve outcome. CT is also used to determine the need for ICP monitoring. In general, ICP should be monitored in all patients with clinical evidence of severe TBI (GCS of 3-8) and any abnormal findings on CT.
Stratifying Injury Severity with CT
CT signs that have been correlated with increased ICP, coma, and death include midline shift greater than 3 to 5 mm (see Figs. 13-4 and 13-5 ), obliterated basal cisterns (see Figs. 13-3, 13-8, and 13-9 ), diffuse hemispheric swelling, SDHs greater than 10 mm in thickness ( Fig. 13-11 ), EDHs greater than 15 mm in thickness (see Figs 13-5A and 13-6B ), SAH, and IVH. Any intracranial mass lesion may warrant surgical evacuation, whether intracerebral hematoma (ICH), hemorrhagic contusion, SDH, or EDH once the size is large enough to cause brain distortion and elevated ICP. SDHs have a higher mortality than acute EDHs, which may seem counterintuitive given that EDHs often have an arterial source. However, the force required to separate the dura from its periosteal attachment to the inner table of the calvarium is considerable, whereas SDHs can continue to increase with little restriction as additional bridging veins tear when stretched beyond their capacity. Significant improvement in mortality is seen when SDHs are evacuated less than 4 hours after injury. Intraparenchymal mass lesions typically result in less mass effect than extraaxial hemorrhagic mass lesions and are less ominous predictors of poor outcome ; contusions or ICHs, however, particularly in elderly patients with low GCS scores, are likely to benefit from prompt intervention. Classification systems relying on mass effect and mass lesions on CT are limited in patients with DAI.
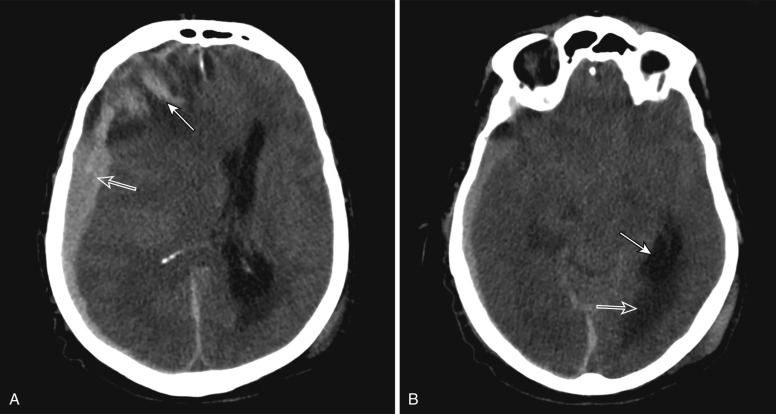
CT Findings in Primary Injury
Cortical Contusions.
Contusions are common, occurring in 43% of closed head injuries, frequently with coexisting coup and contrecoup injuries, and can be multiple or solitary. Cortical contusions represent bruising and laceration of the brain and primarily involve gray matter. Contusions are often based at the cortical surface and extend toward the center of the brain. On noncontrast CT, contusions appear as peripheral low-attenuation areas with loss of gray-white differentiation, reflecting the combination of cytotoxic edema and vasogenic edema involved. In most cases, some hemorrhage is present at MRI, but this may not be detectable at first with CT. When contusions are nonhemorrhagic on MRI, they may also be difficult to appreciate on CT, with subtle areas of low-density edema ( Fig. 13-12 ). Hemorrhagic contusions may take the form of multiple areas of microhemorrhage interspersed with low density, giving a heterogeneous “salt-and-pepper” appearance (see Fig. 13-3A ), or they may appear as more coalescent areas of hemorrhage, giving a more uniformly hyperdense appearance (see Fig. 13-7B ). As the injury progresses, small hemorrhages may coalesce into larger hematomas.
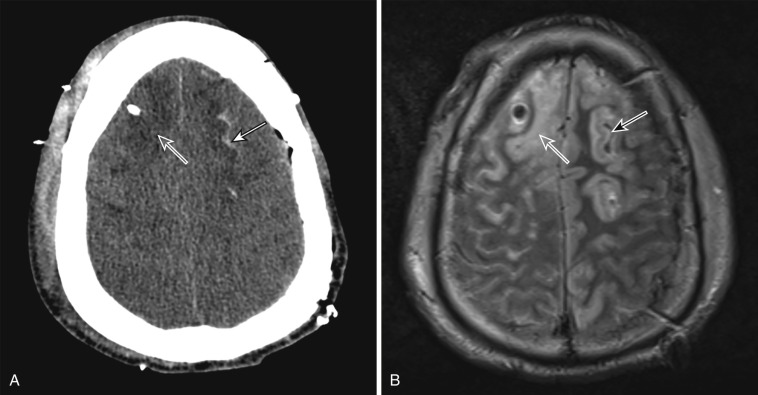
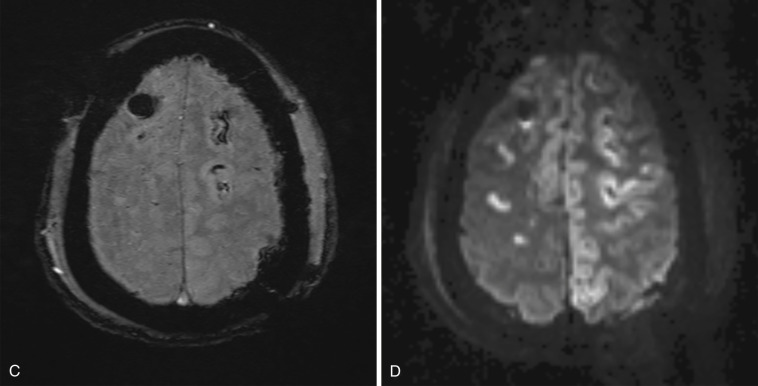
Intracerebral Hematoma.
Whereas ICHs can result from coalescent hemorrhage in contusions ( Fig. 13-13 ), deeper lesions often result from shear-induced hemorrhage and rupture of small intraparenchymal vessels. Unlike contusions, these often occur in areas of otherwise normal-appearing brain and initially have less surrounding edema. These often occur within frontotemporal white matter, sparing the cortex. Deeper lesions may occur in the basal ganglia, but bleeding in this area may also suggest a possible hypertensive hemorrhage. ICHs can be a marker of concurrent DAI even when typical hemorrhagic foci at the gray-white matter junction are absent on CT ( Fig. 13-14 ).
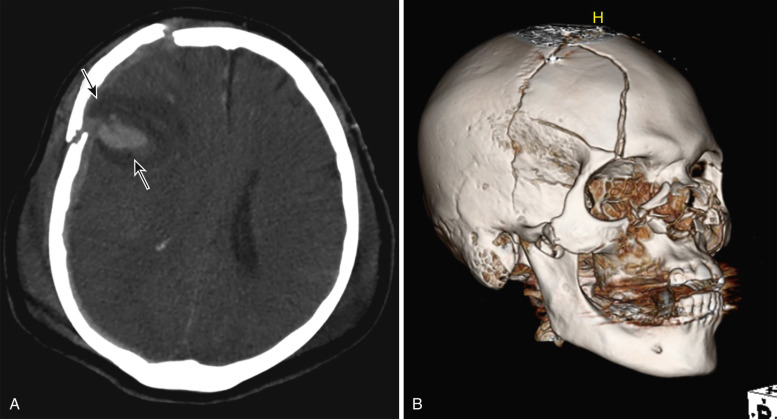
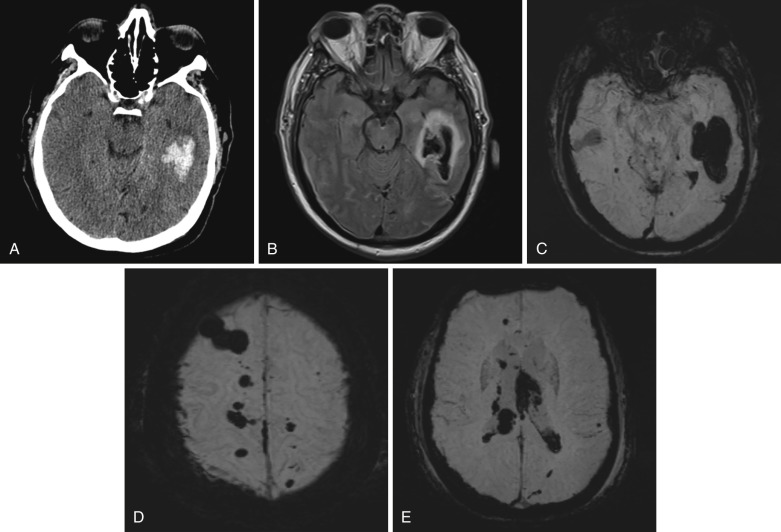
Diffuse Axonal Injury.
DAI is thought to be present in over 50% of severe head injuries and in more than 85% of severe head injuries resulting from motor vehicle accidents. The key point to remember is that DAI is more the rule than the exception in severe TBI patients. DAI presents with the classic CT findings of petechial hemorrhage (an indirect sign of axonal injury) in only 10% to 50% of cases ( Fig. 13-15 ). DAI can be appreciated on CT when small hemorrhagic foci are evident, typically occurring at the gray-white junction ( Fig. 13-16 ) and, in more severe cases, in the corpus callosum complex, which includes the septum pellucidum, fornix, and choroid. Hemorrhagic DAI can also occur in the periventricular white matter at the corner of the frontal horns and in the hippocampal and parahippocampal areas, internal capsule, and cerebellum ( Fig. 13-17 ). Common areas of hemorrhage should be examined very thoroughly for minimal lesions.
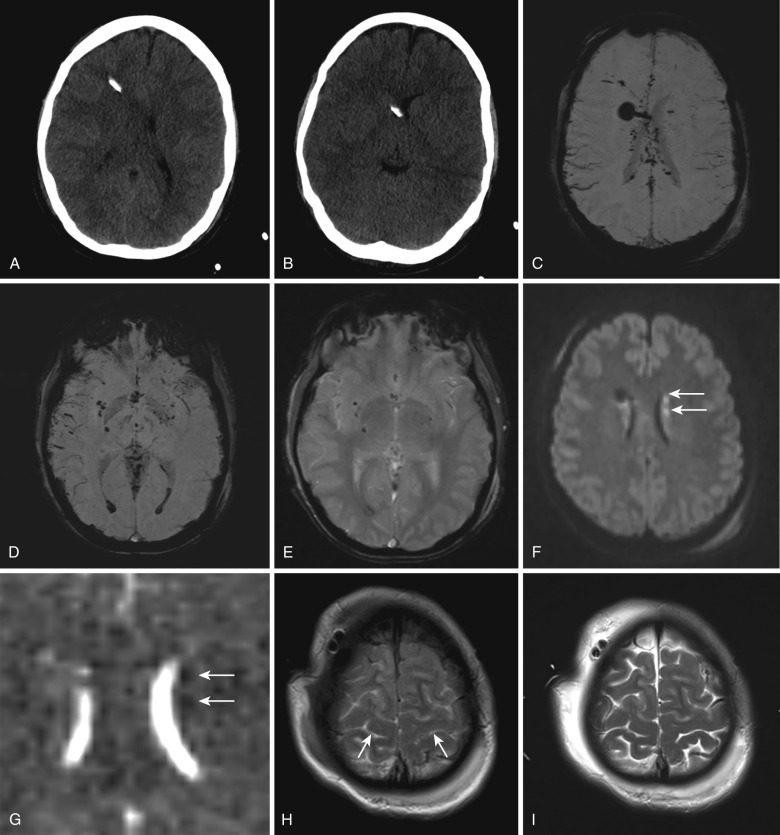
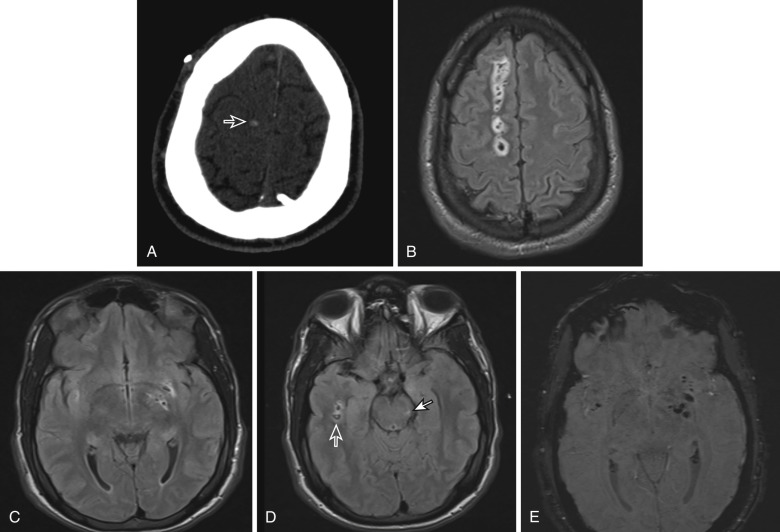
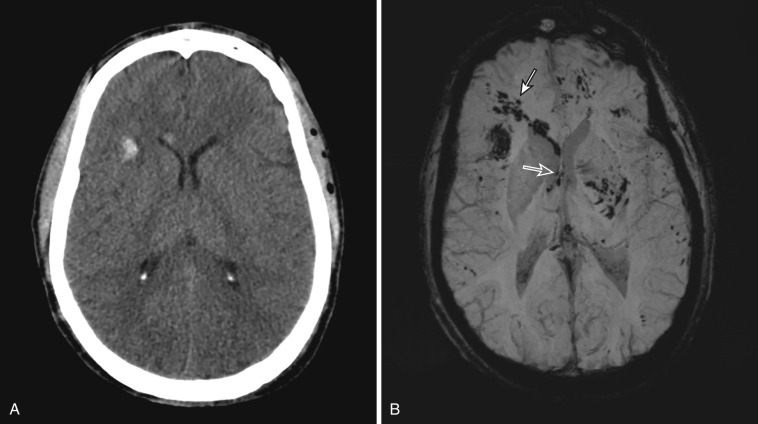
Subdural Hematoma.
In general, the incidence of SDH and EDH is higher when skull fractures are present. SDHs occur along the convexities, tentorium, and falx in descending order of frequency. SDHs follow dural reflections, spreading out along the anterior or posterior falx cerebri and typically the supratentorial aspect of the tentorium cerebelli ( Fig. 13-18 ), which may be symmetric or appear as asymmetric high density ( Fig. 13-19 ). Unlike EDHs, SDHs are not restricted by suture lines. SDHs are usually described as being crescent shaped, in contradistinction to EDHs, which dissect between the dura and inner table, resulting in a biconvex (“lentiform”) appearance. The density of acute SDH is high because of clot formation and retraction (see Fig. 13-7A ). Density is highest in the first week after injury and then gradually decreases with protein degradation and liquefaction. In acute SDHs, heterogeneity can result from active bleeding (see Fig. 13-13A ). SDHs may appear isodense to brain parenchyma in the acute setting if there is severe anemia or trauma-induced coagulopathy or when there is admixture with subarachnoid fluid crossing through an arachnoid tear ( Fig. 13-20 ). Diffuse underlying cortical swelling is common with SDH ; 85% of cases of diffuse swelling and bulk enlargement of a cerebral hemisphere are associated with SDHs.
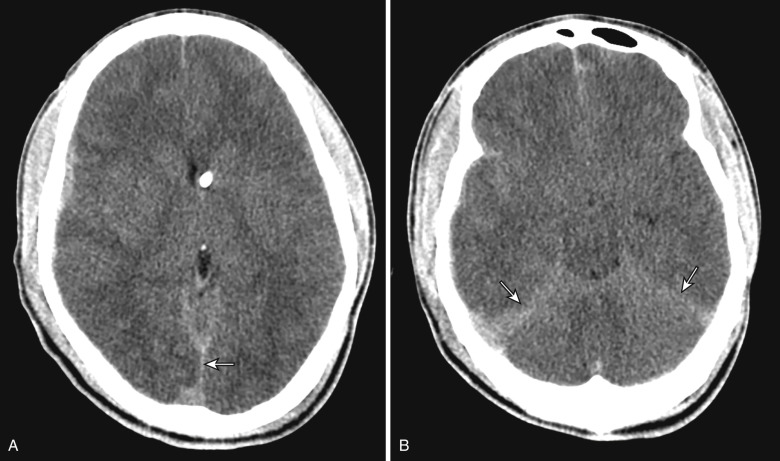
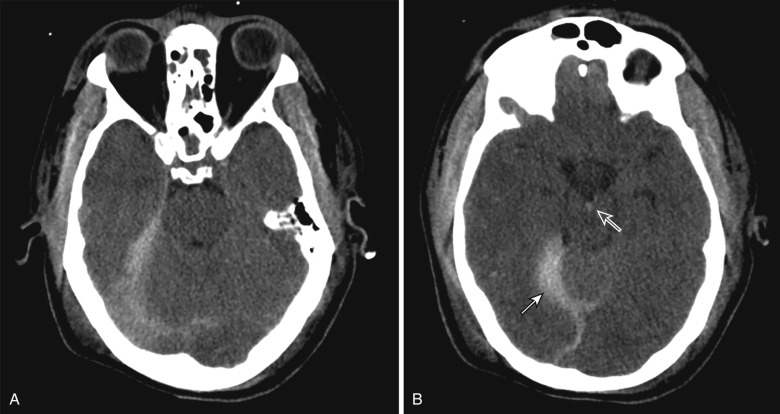
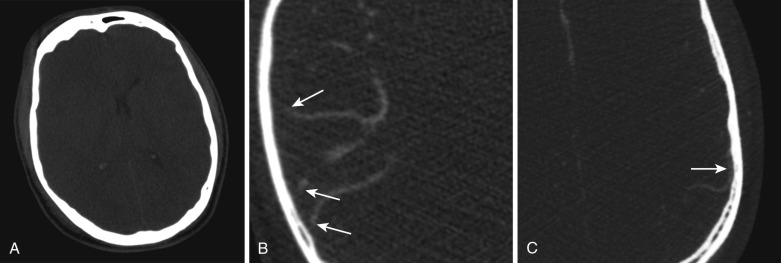
Epidural Hematoma.
EDHs are relatively uncommon, occurring in approximately 4% of head trauma patients. EDHs mostly occur in young adults. In the elderly, the dura is more strongly affixed to the skull, and in children, plasticity of the calvarium mitigates against EDH. In 91% of EDHs, a skull fracture is seen on CT (see Fig. 13-5 ). EDH typically occurs at the site of impact (coup site) in contradistinction to SDH. As EDHs dissect along the tightly adherent inner table and dura, the shape formed by the leading edges is well defined. As with SDH, mixed density may indicate active bleeding. Areas of often central and irregular low density within hyperdense hematoma, representing admixture of liquid blood and fresh clot, has been termed swirl sign. Low-density liquid blood can also occur eccentrically or peripherally (see Figs. 13-5 and 13-6 ). Low-density liquid blood with an EDH is associated with massive hemorrhage and poor outcome. Unlike SDHs, EDHs cross dural reflections. EDHs usually do not cross suture lines unless the sutures themselves are disrupted or diastatic ( Fig. 13-21 ). The sagittal sutural line is an exception because the dura is not as tightly attached to the periosteum as it is elsewhere ( Fig. 13-22 ). Occipital EDHs, often venous in origin, can extend both across the midline and above and below the tentorium ( Fig. 13-23 ), whereas SDHs are restricted by dural reflections ( Fig. 13-24 ). Venous EDHs may cause the dural sinuses to be displaced away from the inner table of the calvarium (see Fig. 13-21C ). Venous EDHs (representing only 15% of EDHs overall) typically occur in three locations: the posterior fossa from torcular injury, middle cranial fossa from sphenoparietal sinus injury, and vertex from injury to the superior sagittal sinus. Venous EDH also occurs in the regions of the transverse and sigmoid sinuses. These EDHs tend to enlarge less quickly than arterial EDHs.
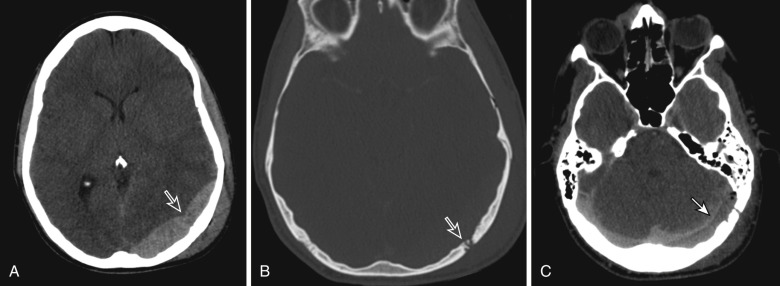
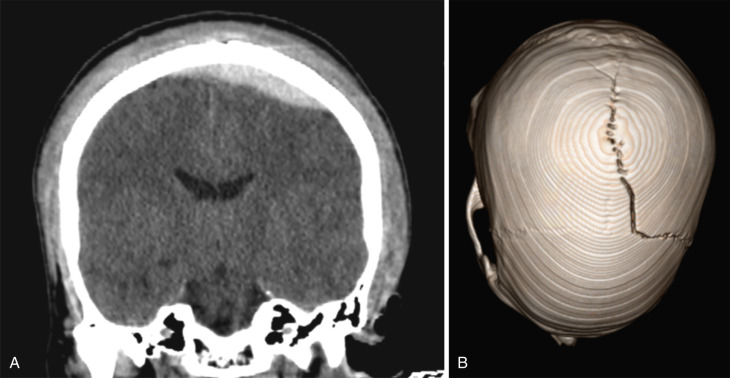
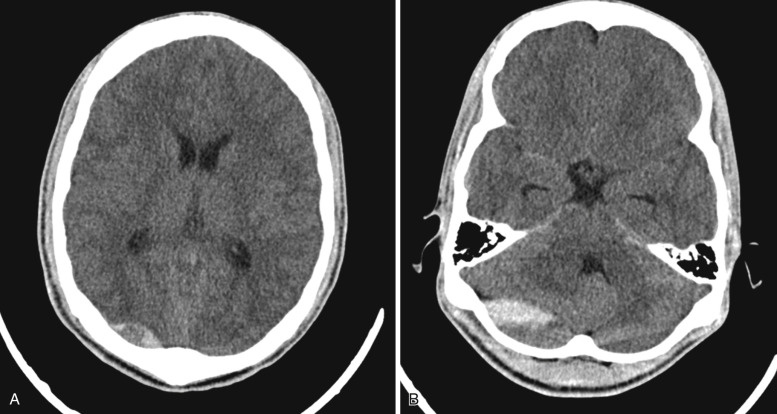
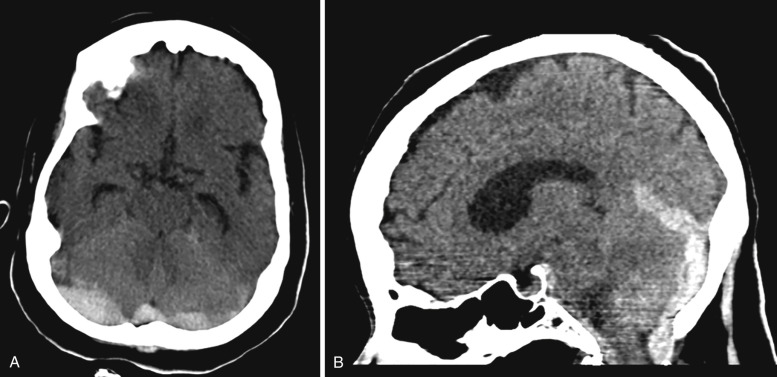
Intraventricular Hemorrhage.
IVH is uncommon, occurring in approximately 3% of newly diagnosed cases of TBI, but it contributes significantly to morbidity and mortality, with half of patients with IVH developing increased ICP, and 10% requiring ventricular drainage. IVH usually appears as a hyperdense blood-fluid level layering dependently in the ventricular system. In small hemorrhages this can be subtle, with punctate foci appearing in the tips of the occipital horns (see Fig. 13-15A ). Occasionally IVH may appear tumefactive as a cast within the ventricle ( Fig. 13-25 ).
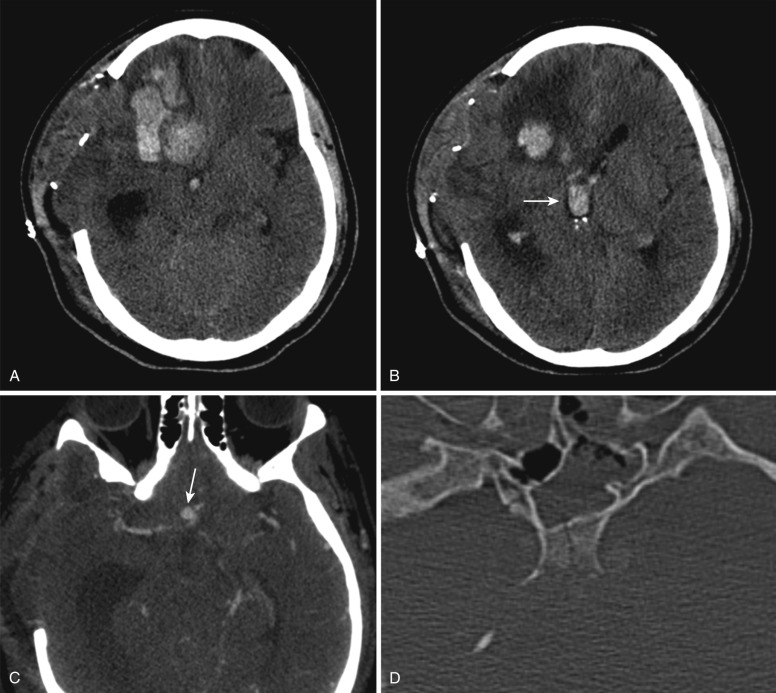
Subarachnoid Hemorrhage.
SAH occurs in 40% of cases of moderate to severe head injury and is usually associated with other types of intracranial hemorrhage. The volume of extravasated blood is rarely as large or extensive as in aneurysmal SAH and more often has a peripheral distribution, sometimes adjacent to areas of cortical contusion. Acute SAH is readily identified on noncontrast CT as linear and serpentine areas of high attenuation extending into and conforming to the cerebral sulci and sylvian fissures without mass effect ( Fig. 13-26 ; see also Fig. 13-12A ). SAH along the convexity, tentorium, or falx can be difficult to differentiate from SDH. Small foci of SAH at the interpeduncular cistern may be easily missed without conscious attention to this area (see Fig. 13-19B ). Although SAH is usually associated with other findings, it may be the sole finding in a minority of cases. In the subacute setting, SAH becomes isodense and may be very subtle; effacement of sulci may be the only imaging clue. The reason for increased mortality is uncertain but is hypothesized to be related to vasospasm.
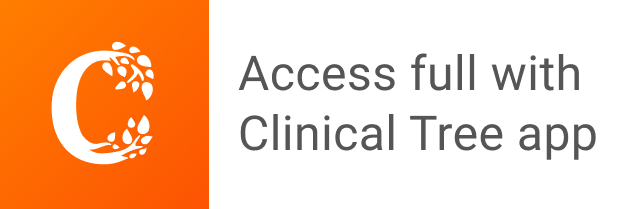