Fig. 45.1
Typical examples of 11C-NMSP microPET imaging in various conditions. Coronal and axial sections of rat brain are shown. White cross markers in the coronal and axial sections indicate the same position in a scan. (a) Typical image of 11C-NMSP microPET in normal rat brain showing high 11C-NMSP accumulation in the striatum. (b) L/N ratio of 11C-NMSP decreased after traumatic brain injury. (c) High accumulation of 11C-NMSP indicated the existence of transplanted DRD2-positive NSCs (From Zhang et al. (2008); with permission)
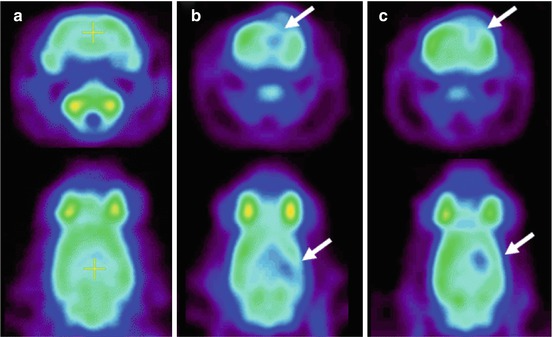
Fig. 45.2
Typical examples of 18F-FDG microPET imaging in various conditions. Coronal and axial sections of rat brain are shown. (a) Typical image of 18F-FDG microPET in normal rat brain. (b) As shown with arrows, the focal traumatic lesion appeared as a hypometabolic area in the right parietal cortex. (c) The regional glucose metabolism of the focal traumatic lesion recovered 14 days after NSCs transplantation (From Zhang et al. (2008); with permission)
PET Imaging of Neuroinflammation
Cellular processes that have received much attention lately are those involved in TBI neuroinflammation. Microglial, astrocyte, and neuron cells resident in the central nervous system begin to react in the acute TBI phase and are subsequent and chronically activated. The role of activated microglia is to serve as the most important antigen-presenting cells and to synthesize inflammatory mediators and complement, which are crucial in the neuroinflammatory cascade after TBI. Microglia functions are very complex, since they have both neurotoxic and neuroprotective roles. Several researchers have suggested that the neuroinflammatory response may explain the great variability in long-term clinical course after TBI. Previous studies in TBI animal models have demonstrated that the inflammatory process may persist for at least a year, especially in the thalamus (Nagamoto-Combs et al. 2007, 2010). Human postmortem studies have also found microglia activation many years after TBI (Gentleman et al. 2004).
The neuroinflammatory response can be studied in vivo with PET using the radioligand 11C-(R)-PK11195 (1-[2-chlorophenyl]-N-methyl-N-[1-methylpropyl]-3-isoquinoline carboxamide), which is a selective marker for activated microglia. (R)-PK11195 binds to the 18 kDa translocator protein (TSPO), expressed in the mitochondria of activated microglia. 11C-(R)-PK11195 PET has previously been used to study neuroinflammation in several neurodegenerative diseases. Chen and Guilarte (2008) published an excellent overview of the use of TSPO as a biomarker for active neuroinflammation in experimental and human studies.
The first studies using 11C-(R)-PK11195 PET in TBI patients have been published recently (Folkersma et al. 2011; Ramlackhansingh et al. 2011). In the paper by Folkersma and coworkers, a patient group in chronic stage with moderate or severe TBI (N = 8) and a control group (N = 7) were studied. 11C-(R)-PK11195 PET and MRI images were acquired 6 months after TBI. 11C-(R)-PK11195 BPND parametric images were generated. To generate a reference tissue input, the authors used supervised cluster analysis (Boellaard et al. 2008). Evaluation of the 11C-(R)-PK11195 BPND was done in the whole brain and regionally. Group comparisons showed a significant increase of whole-brain 11C-(R)-PK11195 BPND in the TBI group. This increase was not only observed in structurally affected brain regions (MRI) but also in apparently normal regions. On the other hand, there was no correlation between TBI severity (GCS) or neurological outcome (GOS) and whole-brain 11C-(R)-PK11195 BPND. Although microglial activation is mostly a diffuse event in TBI, brain regions showing significant increases of 11C-(R)-PK11195 BPND were the left and right frontal lobe, left and right thalamus, left parietal lobe, right temporal lobe, hippocampus and putamen, midbrain, and pons. An interesting finding was that 11C-(R)-PK11195 BPND was maximal in the thalamus in six out of eight patients.
The study by Ramlackhansingh and coworkers (2011) investigated whether the inflammatory response persists in patients with chronic TBI and if this response was related to structural abnormalities and cognitive dysfunction. This paper included a group of patients with moderate to severe chronic TBI (N = 10). Five patients had focal damage visible in MRI images, while the other five did not show abnormalities. 11C- (R)-PK11195 PET was performed on all patients at least 11 months after TBI. Like in the previous study, 11C-(R)-PK11195 BPND parametric images were generated using supervised cluster analysis. Volumetric MRI and DTI were done to evaluate focal damage and the disruption of WM. Cognitive function was also evaluated. Group comparisons showed that 11C-(R)-PK11195 BPND was increased in the thalami, putamen, occipital cortices, and posterior limb of the internal capsules in the patient group compared with controls (Fig. 45.3). Unlike the study by Folkersma and coworkers, they found no increase in 11C-(R)-PK11195 BPND at the original site of focal brain injury, which is probably due to the different intervals which had elapsed after TBI in both studies. In the patient sample, a positive correlation was observed between 11C-(R)-PK11195 BPND in the thalamus and the degree of cognitive impairment. 11C-(R)-PK11195 BPND increase was not associated to structural damage found by volumetric MRI and DTI or the time elapsed after TBI. Like in the article by Folkersma, persistent microglia activation in chronic TBI patients was confirmed especially in subcortical regions. Taking into account the long intervals after injury in the patient sample, this paper also suggests that therapeutic interventions can be beneficial even for a long time after TBI.
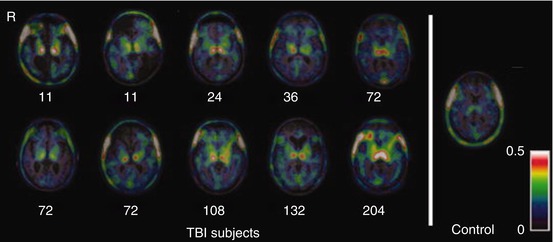
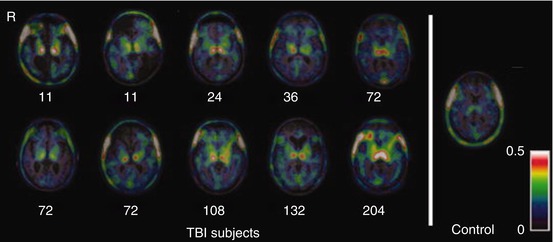
Fig. 45.3
Chronic microglial activation is present following traumatic brain injury (TBI). Overlay images of the transverse T1 magnetic resonance imaging at the level of the thalamus superimposed with 11C-(R)-PK11195 (PK) images of all TBI subjects and a representative control subject. Numbers indicate time in months from the time of TBI to positron emission tomography scanning. Images illustrate the greater binding of PK in the thalami of all TBI subjects. R right (From Ramlackhansingh et al. (2011); with permission)
Although longitudinal studies are required in larger patient samples, the results of both studies indicate that 11C-(R)-PK11195 PET could become an attractive method for detecting secondary damage after TBI and could serve as guide for evaluating interventions directed toward manipulating the inflammatory cascade.
In recent years, there have been great efforts to develop other selective radioligands for neuroinflammation in order to overcome the limitations of 11C-(R)-PK11195 (Chauveau et al. 2011; Antunes et al. 2012). Among these limitations are the high level of nonspecific binding; the low signal to noise ratio, which renders quantification difficult; and the fact that it is labeled with 11C, which limits its use to medical institutions that have a cyclotron.
45.3 Single-Photon Emission Computed Tomography (SPECT)
Perfusion SPECT using 99mTc-hexamethyl-propyleneamine oxime (HMPAO) or 99mTc-ethylene cysteine dimer (ECD) has been extensively used in TBI. Reviews of this neuroimaging modality have appeared regularly in the last years (Davalos and Bennett 2002; Cihangiroglu et al. 2002; Belanger et al. 2007; Tikofsky 2010; Tong et al. 2011). These reviews coincide in pointing out that perfusion SPECT has high negative predictive value during the acute phase in mild TBI. They also agree that perfusion SPECT, like 18F-FDG PET, is more sensitive than CT for identifying abnormalities in TBI during the first hours, detecting them in very early stages, when CT (or MRI) scans may still be negative. Abnormalities detected by perfusion SPECT are more extensive than those observed by structural neuroimaging. A recent study shows that the combination of structural neuroimages, such as CT, with perfusion SPECT using 99mTc-ECD is useful to determine the extent and severity of TBI lesions and tissue viability in core, edema, and perilesional tissue (Pifarré et al. 2011). Figure 45.4 shows CT and SPECT perfusion imaging of a patient with left frontal primarily brain trauma.
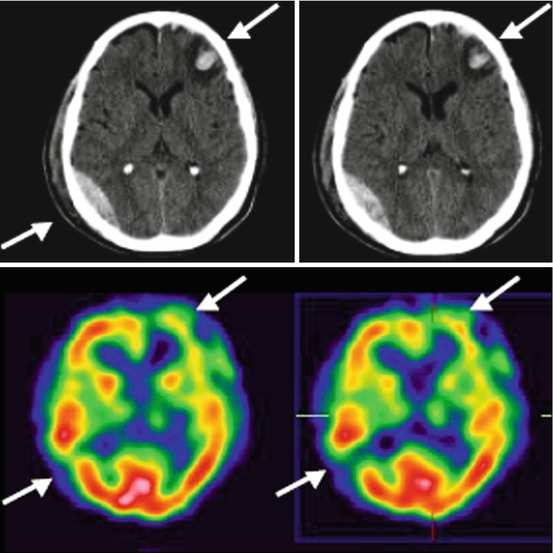
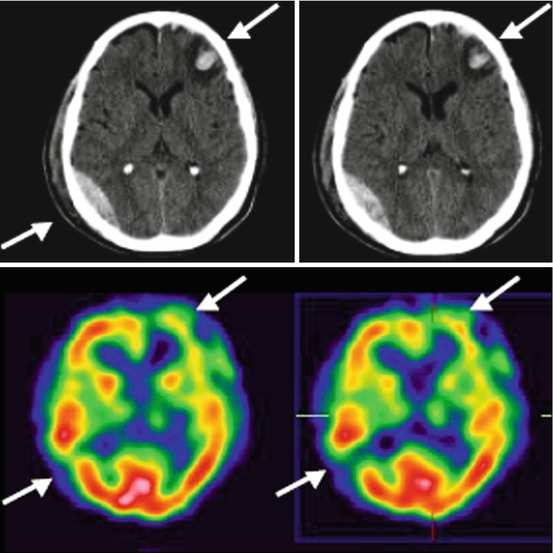
Fig. 45.4
CT and SPECT perfusion imaging of a patient with left frontal primarily brain trauma. In the hemorrhagic lesion seen on the CT in the right posterior temporal region, SPECT images show an absence of perfusion (From Pifarré et al. 2011)
Furthermore, using SPECT it is possible to study cell-specific processes related to TBI pathophysiology. Studies combining 123I-2-β-carbomethoxy-3-β-(4-iodophenyl) tropane (β-CIT) and 123I-iodobenzamide (IBZM) found nigrostriatal dysfunction in TBI patients, although the striatum was structurally relatively preserved, suggesting these studies may be useful in the evaluation of therapies directed toward reducing parkinsonian symptoms in TBI patients (Donnemiller et al. 2000). Another very recent animal study used N′,N′-diethyl-6-chloro-(4′-[123I]iodophenyl)imidazo [1,2-a]pyridine-3-acetamide (123I-CLINDE) for the in vivo monitoring of neuroinflammation by SPECT (Mattner et al 2011). 123I-CLINDE is a highly specific radioligand for TSPO. A recent perfusion SPECT activation study investigated cognitive fatigue mechanisms in patients with mild TBI (Hattori et al. 2009). In this study, it was possible to show that there is frontocerebellar dissociation in patients with mild TBI that may explain cognitive impairment and cognitive fatigue in the chronic phase.
Technological advances in SPECT detector systems, hybrid SPECT–CT, continuous development of new gamma-emitting radioligands, and the application of modern methods of image analysis make the SPECT technique a valid alternative for the study of TBI, both for clinical practice and for research. Nevertheless, like 18F-FDG PET, evidence-based imaging studies are required to demonstrate its incremental validity in TBI. The main advantage of SPECT is that it is much less costly and is still more widely available on a worldwide scale in comparison to PET.
45.4 Conclusions
In the acute TBI phase, 18F-FDG PET seems to be more useful and has prognostic value in patients whose neurological state cannot be explained by structural neuroimages. However, evidence-based imaging studies are necessary to demonstrate the incremental validity of 18F-FDG PET together with cost-effectiveness analysis to appropriately compare 18F-FDG PET with other neuroimaging techniques. Whichever the results of these studies, 18F-FDG PET and 15O2-PET will remain very valuable tools for research of the complex and not yet fully understood pathophysiology of acute-phase TBI. In chronic TBI patients, most of the 18F-FDG PET studies coincide in indicating a diffuse cortical–subcortical pattern of hypometabolism associated with cognitive impairment, even in patients without evident structural abnormalities. Preliminary studies also suggest the usefulness of 18F-FDG PET for the evaluation of different therapeutic approaches for improving cognitive function in chronic TBI. In recent years, interest in studying cell-specific processes involved in TBI pathophysiology with PET is growing. An example of such studies is the use of radioligands as markers of neuroinflammation. Neuroinflammation imaging could become very attractive for detecting secondary damage after TBI, possibly with higher sensitivity than 18F-FDG PET. Furthermore, it could serve in the evaluation of different therapeutic approaches. Finally, SPECT is a valid alternative for the study of TBI. However, as with PET, evidence-based imaging studies are required to demonstrate its incremental validity. SPECT is much less expensive and more widely available on a global scale.
References
Alavi A (1989) Functional and anatomic studies of head injury. J Neuropsychiatry Clin Neurosci 1:S45–S50PubMed
Alavi A, Mirot A, Newberg A et al (1997) Fluorine-18-FDG evaluation of crossed cerebellar diaschisis in head injury. J Nucl Med 38:1717–1720PubMed
Antunes IF, Doorduin J, Haisma HJ et al (2012) 18F-FEAnGA for PET of β-glucuronidase activity in neuroinflammation. J Nucl Med 53:1–8. doi:10.2967/jnumed.111.096388 CrossRef
Bassett DS, Bullmore ET (2009) Human brain networks in health and disease. Curr Opin Neurol 22:340–347PubMedCentralPubMedCrossRef
Boellaard R, Turkheimer F, Hinz R et al (2008) Performance of a modified Supervised cluster algorithm for extracting reference region input function from [11C](R)-PK11195 brain PET studies. IEEE Nucl Sci Symp Conf Rec 5400–5402
Bullock R, Chestnut RM, Clifton G et al (2007) Guidelines for the management of severe traumatic brain injury, 3rd ed. J Neurotrauma 24(Suppl 1):S26–S31
Chen MK, Guilarte TR (2008) Translocator protein 18 kDa (TSPO): molecular sensor of brain injury and repair. Pharmacol Ther 118:1–17PubMedCentralPubMedCrossRef
Cihangiroglu M, Ramsey RG, Dohrmann GJ (2002) Brain injury: analysis of imaging modalities. Neurol Res J24:7–18CrossRef
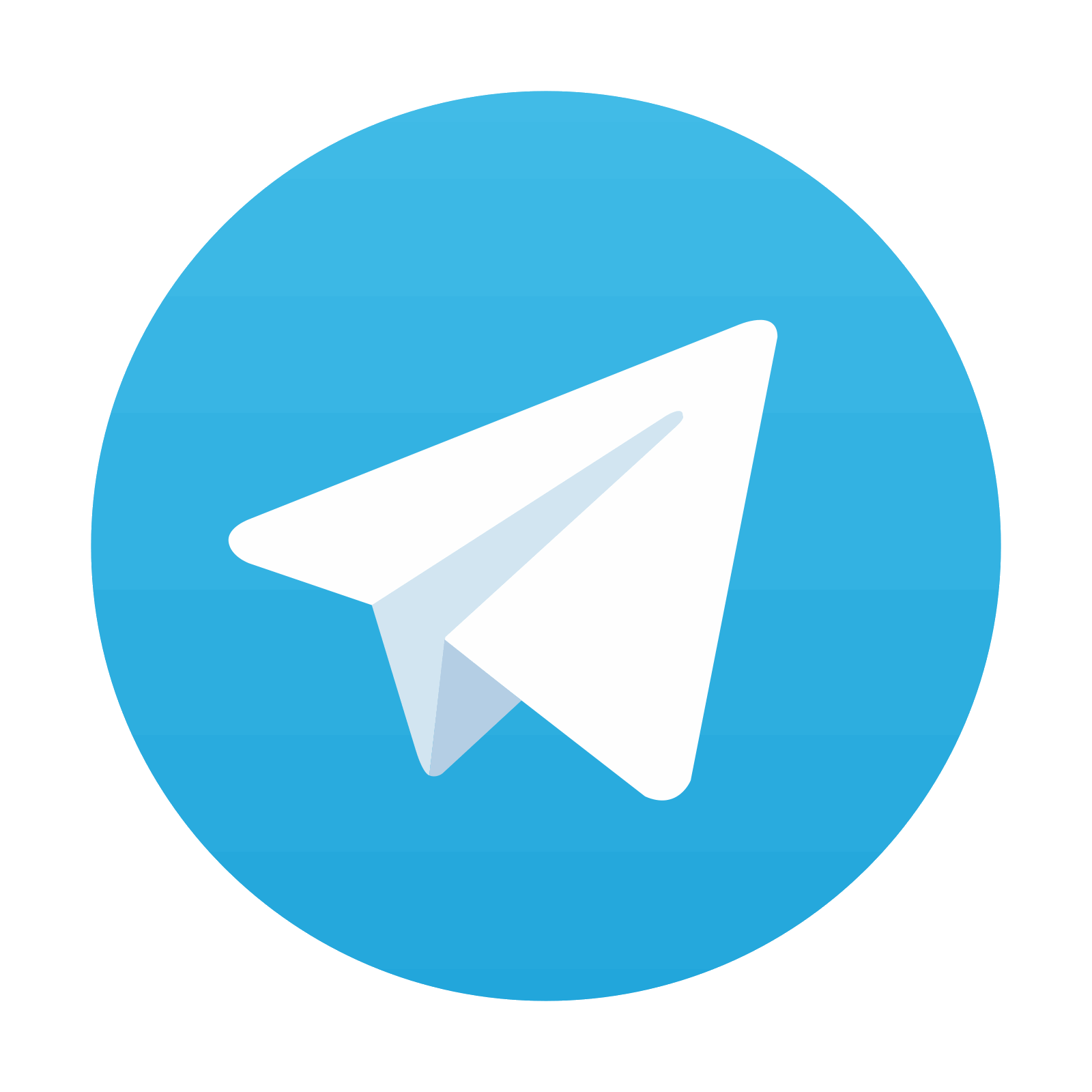
Stay updated, free articles. Join our Telegram channel
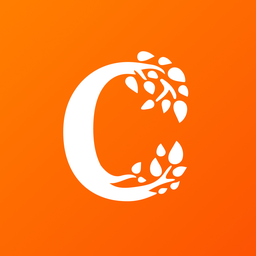
Full access? Get Clinical Tree
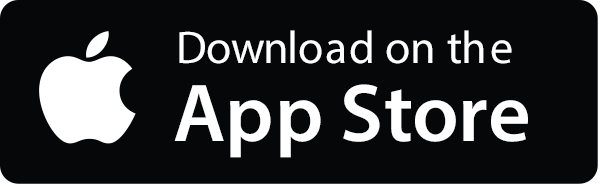
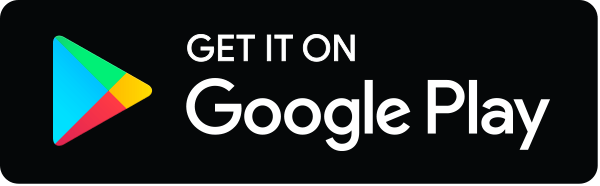