Abstract
Prior to the widespread use of magnetic resonance imaging (MRI), the diagnosis of acute traumatic spinal cord injury (SCI) was largely based on clinical history, physical examination, plain film X-ray, CT scan, and CT myelography. Prognosis was loosely based on the severity of injury whereby individuals presenting with severe injuries were deemed unlikely to recover, and those with mild injuries were thought to recover to a greater extent. The implementation of clinical MRI units from the 1980s to present has offered clinicians an opportunity to view the damaged spinal cord. In this chapter we will (1) describe the meaning of different MRI signal characteristics relative to the pathobiology of SCI as elucidated in animal imaging models, (2) describe how quantitative measurements of spinal cord compression can be used as a tool to determine the degree of spinal cord damage in a patient, and (3) describe how intramedullary MRI signal characteristics can been used as a diagnostic and prognostic tool.
Keywords
Acute spinal cord injury, Clinical examination, Magnetic resonance imaging, Prognosis, Trauma
1.3A.1
Introduction
Prior to the 1980s a diagnosis of trauma to the spinal cord was largely inferred based on either X-rays showing misalignment of the spinal column or myelography whereby interruption of the flow of contrast medium in the cerebral spinal fluid (CSF) space indicated impingement of the spinal cord. With the widespread adoption of MRI came the visualization of the spinal cord itself, and along with it a revolution in the diagnosis and treatment of traumatic spinal cord injury. It wasn’t long after the implementation of MR imaging that numerous research groups began to study the ability of MRI to determine both neurological function and prognosis following spinal cord injury. Numerous studies were undertaken with an aim of understanding the limitations of MRI to aid clinicians in treating their patients. These studies, reviewed and summarized here, introduced a host of new questions to the field such as the meaning of different signal characteristics with respect to pathological changes in the spinal cord tissue and the ability of these signal characteristics to yield meaningful clinical information such as the degree of damage to the spinal cord in terms of neurological function and the potential prognostic implications.
The advantages of being able to both determine neurological function and predict prognosis after acute traumatic spinal cord injury by reviewing MRI scans are numerous. While determining function and prognosis may seem beyond the limits of structural MR imaging, significant progress has been made in this direction. Benefits include distinguishing subclasses of patients that would benefit from different treatment options; for example, persons with severe spinal cord compression may benefit most from urgent decompressive surgery. Others, with no spinal cord compression, may benefit from delivery of neuroprotective agents, either systemically or locally. Accurate diagnostic and prognostic information could be conveyed to patients, family members, and the rehabilitation team such that efforts can be coordinated to plan and execute the best rehabilitation strategy.
Other modalities have certainly been used to predict prognosis following spinal cord injury, each with its own limitation. Physical examination, electrophysiology, computed tomography, and myelography have been studied. Of these, neurological examination has maintained its position as the gold standard for assessing patients both in the acute stage and at long-term follow-up. There are however two factors that make an alternate test worth seeking. The first is that patients who suffer from traumatic spinal cord injury often have other associated injuries, are intoxicated or are medically unstable making a thorough examination impossible. The second is that a neurological examination taken immediately after injury is often not a good indicator of prognosis.
In this chapter we aim to convey three messages. The first will describe the meaning of different MRI signal characteristics relative to the pathobiology of spinal cord injury as elucidated in animal imaging models. We will place an emphasis on animal imaging models that use MRI as a prognostic tool after traumatic spinal cord injury. The second message is to describe how quantitative measurements of maximum spinal cord compression (MSCC) and maximum canal compromise (MCC) can be used as a tool to describe the degree of spinal cord damage in a patient. The third message of this chapter is to describe how intramedullary MRI signal characteristics have been used as a diagnostic and prognostic tool. To accomplish this, we have conducted a meta-analysis of published papers and constructed receiver operator characteristics to describe the sensitivity and specificity of different MR signal characteristics.
1.3A.2
Message 1: Animal Models That Link Pathology of Acute Spinal Cord Injury to MR Signal Characteristics
Table 1.3A.1 summarizes the animal literature regarding the use of MRI as a prognostic tool in spinal cord injury. Of the eight animal studies identified, five were performed in a rat model and three in a mouse model. Each study involved a surgical procedure to induce spinal cord injury followed by serial clinical examinations and MRI studies. Attempts were then made to correlate clinical status with MRI findings.
Investigators (y) | Species | Injury Model | MRI a Measure | Conclusion |
---|---|---|---|---|
Bilgen et al. | Rat | Contusion injury to mid-thoracic cord | Gd b -enhanced T1 weighted images | T1 contrast enhancement correlates with the degree of NR f |
Narayana et al. | Rat | Contusion injury to mid-thoracic cord | T1, T2, and density-weighted images | Return of gray-white differentiation correlates with NR |
Deo et al. | Rat | Contusion injury to mid-thoracic cord | Diffusion tensor imaging | DTI c metrics do not consistently correlate with NR |
Stieltjes et al. | Mouse | Spinal cord transection (80%) at the mid-thoracic level | Manganese-enhanced MRI images | Manganese enhanced MRI correlates with NR in animals treated with novel therapeutic agents |
Bilgen et al. | Mouse | Contusion injury to mid-thoracic cord | High resolution (9.4 T) images | Differential MRI characteristics did not correlate with NR |
Nossin-Manor et al. | Rat | Hemi-crush injury to mid-thoracic cord (mild vs. severe) | Diffusion-weighted MRI (high b-value, q-space) | Novel DWI d characteristics correlate with NR |
Nishi et al. | Mouse | Contusion injury to mid-thoracic level (mild, moderate, severe) | T1 and T2 images at 7 Tesla | Lesion volume (T1 images) correlates with NR |
Mihai et al. | Rat | Contusion injury to cervical cord (unilateral C5) | T1, T2, and proton density images (±Gad e ) | Hypodense T1 signal and lesion length correlates with NR |
Six of the eight studies showed a positive correlation between prognosis and MRI characteristics. Two of the eight studies showed no such correlation. These discrepancies can easily be explained by the fact that each of these studies examined novel ways of imaging the injured animal spinal cord. The negative studies examined the use of diffusion tensor metrics and high magnetic field strength (9.4 T) on recovery. Establishing these novel methods in an animal model must take place prior to using them to predict either neurological function or prognosis.
Of the studies that showed a positive correlation, two focused on vascular effects, two focused on neuronal structure and function, and two simply reported MRI characteristics as they relate to functional recovery.
1.3A.2.1
Vascular Effects
Bilgen et al. focus on the disruption of the blood–spinal cord barrier (BSCB) following injury. The degree of MR contrast directly correlated with neurological outcome (higher contrast uptake relates to poor outcome). Followed over time, neurological improvement comes at a point when contrast uptake into the lesion diminishes. This suggests that reformation of the BSCB is important for regain of neurological activity. In a similar longitudinal study that combines clinical, MRI, and histological data, the authors demonstrate that spontaneous recovery occurs between two and eight weeks. As neurological function returns, there is a gradual return of gray-white differentiation adjacent to cord contusion (noted by areas of hypo- and hyperintense T2W images).
1.3A.2.2
Neuronal Structure and Function
Two of the animal studies investigated neuronal function via MR imaging after injury. Stieltjes et al. used manganese (a surrogate marker for neuronal activity) as a contrast agent. They detected changes in neuronal structure and function following injury and compared this with recovery. Using an antibody to CD95 ligand, a neutralizing antibody shown to prevent apoptotic cell death and promote neuronal recovery in animal models, the authors compared functional tests with MRI findings. There was a strong correlation between manganese uptake, especially caudal to the level of injury, and functional recovery after treatment with the antibody. Also investigating neuronal function, Nossin-Manor et al. used a novel MRI sequence (high b-value q-space diffusion MRI) that provides information about the integrity of white matter tracts. The authors subjected rats to either a mild or severe hemi-crush injury to the mid-thoracic cord and followed the evolution of injury with both MRI and behavioral testing. Subsequent histological analysis was carried out. Functional recovery occurred in the mild injury group but not in the severe injury group. When comparing the mild and severe injury group at five days post injury, the diffusion weighted imaging (DWI) characteristics were similar at the lesion site. As the distance from the injury site increased, the differences in DWI characteristics became apparent between the mild and severely injured groups. Over the course of six weeks, there was improvement in the DWI characteristics of the mild injury group but not the severe injury group. The improvement in MRI characteristics correlated with neurological recovery.
1.3A.2.3
MRI Characteristics as they Relate to Functional Recovery in Animals
Both Nishi et al. and Mihai et al. carried out longitudinal studies that involved spinal lesions, with varying degrees of force, followed by clinical testing, MRI studies, and histological analysis. Ex vivo MRI revealed lesion volumes that correlated with both the force of injury and behavioral improvement following injury. In vivo MRI revealed cord swelling (increased cord volume), cord edema (hypointense T1 and hyperintense T2 signal), and lesion length to be the most valuable parameters as these were highly correlated to behavioral outcomes and histopathological characteristics of the lesion.
As a final point, Bilgen et al. used a contusion model to the mid-thoracic spinal cord and followed the injury with serial MRI scans, behavioral testing, and histological analysis. Despite receiving the same contusion injury, two different MRI injury patterns were noted in the animals: focal and diffuse. The neurological recovery in each of these subgroups was not statistically different. The authors attribute the different MRI characteristics to a vascular and inflammatory phenomenon. In this sense, the different MRI characteristics did not reflect different neurological improvement.
Each of the previous eight studies was carried out using a similar injury model and a wide variety of MR imaging techniques. While each study focused on unique aspects of spinal cord injury pathophysiology, ranging from vascular phenomena to neuronal function, all attempted to correlate behavioral improvement with imaging characteristics.
1.3A.3
Message 2: Quantitative MSCC and MCC
In an attempt to delineate the precise cause of neurological dysfunction following acute, traumatic spinal cord injury, researchers have divided the temporal sequence of destructive events into primary and secondary injury. Primary injury refers to the destructive forces that directly damage the neural structures such as the shear force tearing an axon or the direct compressive force occluding a blood vessel resulting in ischemia. These destructive primary mechanisms not only result in instantaneous damage to neurons and blood vessels but also initiate a cascade of cellular mechanisms that result in ongoing damage to the neural structures, termed secondary injury. In fact, in cases of ongoing primary injury, for example in the setting of a fracture dislocation where the bony spinal column is displaced and physically pushed up against the spinal cord, these cellular mechanisms are thought to be locked into the “on” position until such physical forces are removed either by closed reduction or surgically. A detailed explanation of the secondary injury hypothesis of traumatic spinal cord injury can be found in the seminal publication by Tator and Fehlings.
In order to account for the ongoing forces of spinal cord compression that may exacerbate the initial injury, the Fehlings research team established a quantitative method for evaluating and reporting spinal canal compromise and spinal cord compression. Through a multicenter study, the authors established the following criteria.
1.3A.3.1
Maximum Canal Compromise
Using mid-sagittal CT reconstructions or MRI images and the respective axial slices, one should identify the level of maximum spinal canal compromise (MCC) and compare this with the normal canal diameter at the mid-vertebral body level above and below the lesion. This is quantified using the formula shown in Figure 1.3A.1 where D i is the anteroposterior canal distance at the level of maximum injury, D a is the anteroposterior canal distance at the nearest normal level above the level of injury, and D b is the anteroposterior canal distance at the nearest normal level below the level of injury.
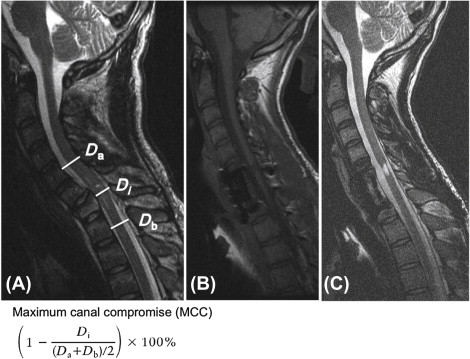
1.3A.3.2
Maximum Spinal Cord Compression
The anteroposterior cord diameter on mid-sagittal and axial T2 MRI images at the level of maximum compression should be compared with the anteroposterior cord diameter at the normal levels immediately above and below the level of injury. If cord edema is present, measurements of normal anteroposterior cord diameter should be made at mid-vertebral body levels just beyond the rostral and caudal extent of the cord edema at the levels where the cord appears normal. These values are quantified using the formula depicted in Figure 1.3A.2 where d i is the anteroposterior cord distance at the level of maximum injury, d a is the anteroposterior cord distance at the nearest normal level above the level of injury, and d b is the anteroposterior cord distance at the nearest normal level below the level of injury.
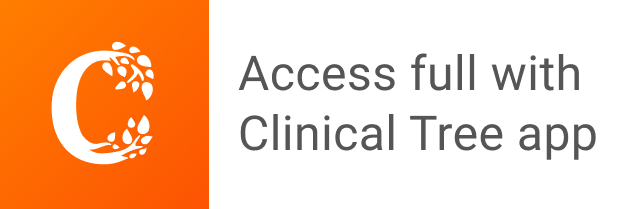