Fig. 6.1
The basic steps in SRS treatment planning
Stereotactic Localization
Because SRS involves the delivery of a very high dose of radiation in either a single delivery or a small number of fractions, it is essential to achieve a high degree of dose conformity and accurate patient positioning. This critical dosimetric requirement is accomplished by stereotactically focusing many convergent radiation beams on the target. By cross-firing a large number of beams, a high dose is delivered in the region of intersection with a rapid falloff in dose outside of the target.
One approach to accurate stereotactic localization is achieved with fixation frames such as the frame shown in Fig. 6.2. On the day of the procedure, this fixation frame is fixed to the patient’s skull under local anesthetic. The frame serves two important purposes. First, it provides a rigid fixation system that ensures that the patient cannot move during the delivery. Secondly, it provides a frame of reference whereby the tumor location can be determined relative to the frame and relative to the delivery unit.
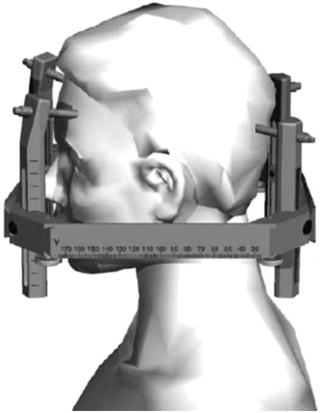
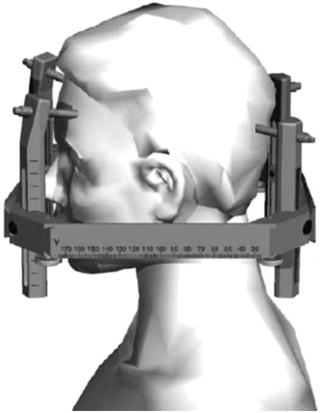
Fig. 6.2
Gamma knife coordinate frame (Photo courtesy of Elekta)
One approach for facilitating the registration of the patient images is to attach a fiducial box (see Fig. 6.3) to the stereotactic head frame before patient imaging. The box includes tubes filled with a high-contrast solution that provides easy to delineate fiducial marks on each of the patient’s images (see Fig. 6.4).
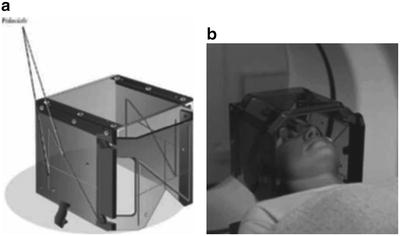
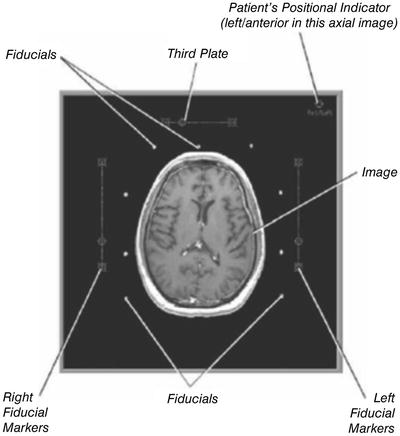
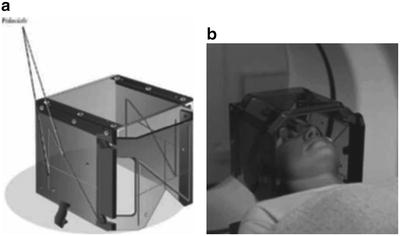
Fig. 6.3
(a) The fiducial indicator box for CT imaging. (b) The patient is imaged with a fiducial box attached to the frame (Photos courtesy of Elekta)
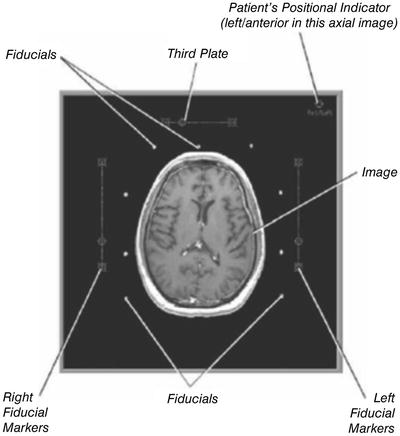
Fig. 6.4
The fiducial marks appear as white dots on this MRI image of a Gamma Knife patient (Photo courtesy of Elekta)
After the imaging is complete, the patient images are loaded into the planning system. The images are registered with respect to the fiducial marks. The markings on the fiducial box provide a coordinate system that makes it possible to precisely determine the position of the treatment volume with respect to the stereotactic head frame.
In addition to the fixation frames described above, frameless SRS techniques have been developed that maintain a highly precise delivery [6–12]. Frameless SRS is typically the preferred option in fractionated radiosurgery where the invasive nature of fixation frames makes them a less viable option. Fractionation radiosurgery, known as stereotactic radiotherapy (SRT), is commonly delivered to patients where the likelihood of late toxicity makes it inadvisable to treat a large target volume in a single high-dose fraction [7, 12–14].
Patient Imaging
The three primary imaging techniques for SRS are MRI, CT, and planar angiography. MRI is an excellent technique for imaging soft tissue contrast. MRI, however, does not provide attenuation coefficients, and must be fused with CT images if one wishes to correct for to make appropriate heterogeneities in the dose calculation. It should be noted that in most MRI units the spatial uniformity of the images degrades as the radius of the images increases. Because most fiducial systems place the fiducial markers at the outermost extent of the MRI image volume, the accuracy obtained in mapping the imaged voxels to stereotactic space can be compromised. This is one of several reasons some radiosurgical practitioners have opted for image fusion technologies as opposed to direct stereotactic MRI scanning (see Fig. 6.5).
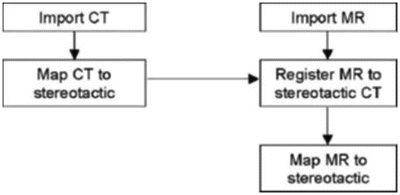
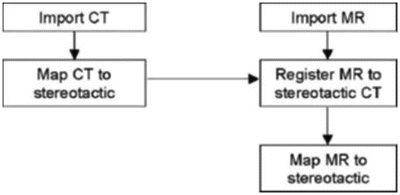
Fig. 6.5
One approach to image registration
CT imaging provides inferior soft tissue contrast as compared with MRI scanning. However, the spatial resolution of CT is extremely reliable. CT also provides attenuation coefficients that make it possible to make accurate heterogeneity corrections in the dose calculation.
Planar angiography is an imaging option that can be used for imaging patients with arteriovenous malformations (AVMs). While plane film angiography was the gold standard for AVM nidus identification, cut films have almost been fully replaced by electronically derived images. For the past few decades, the primary mode of electronic image acquisition has been the image intensifier. While this device is known for its excellent image contrast, it is equally known for its poor spatial accuracy. Most image intensifiers have multiple levels of image distortion. More recently, solid-state image acquisition systems have been developed. These systems have all but eliminated concerns regarding spatially nonuniform images.
An additional problem with angiography is that while plane film angiography can provide images in multiple planes, the basic image set that the clinician has to work with is two dimensional. This two-dimensional image dataset can lead to inaccuracies in the target definition. It is for this reason that most targeting for vascular radiosurgery targets are obtained either through CT angiography, MRI angiography, or both.
Registering the Images
The general planning procedure starts with the acquisition of three-dimensional image information and the import of patient images into the treatment-planning system via a computer network or data storage media. On the planning computer, these images are typically displayed in two-dimensional slices along the axial, sagittal, or coronal planes [15].
A screen capture from the GammaPlan system is shown in Fig. 6.4 that illustrates the process of aligning the fiducial marks in the SRS planning system. The planning system must be able to accurately identify the fiducial marks and have internal mechanisms for validating their consistency. Once the fiducial markers are identified, the patient anatomy shown on the images is placed in the coordinate system defined by the stereotactic localization device.
Some systems use fiducial markers that fully define the stereotactic reference system while others require specific information from the scanner. For example, a fully defined system does not require information on the axial slice position. This is because it can derive this information from the actual scan image. Consequently, this approach eliminates the need for QA measurements to guarantee accurate scanner information such as linearity of table movement or degree of gantry tilt.
Frameless systems also need to be able to map the three-dimensional dataset into a rigid and definable coordinate system. To achieve this, stereotactic systems have been designed that incorporate surrogate fiducial markers into the CT or MRI scans. These markers can also be referenced at the time of therapy through mechanical, electrical, or optical means. A critical feature of frameless systems is the ability to demonstrate that the reference can in fact be reliably and precisely fixed to the patient. Many systems offer testing only at the time of design and development. These tests are usually at the hands of the developer, an expert with extensive experience. It is critical for a repeat fixation system to also provide a methodology of testing its accuracy for each patient.
Shows the RadioCameras™ Treatment Guidance System (Varian, Palo Alto, CA). This system provides patient-specific statistics on the device’s ability to be precisely reapplied.
Two basic approaches are used in the registration of images. The first is to use direct stereotactic MRI and CT scanning. In this procedure, fiducial markers are incorporated into both image datasets and each dataset is independently mapped into stereotactic space. In the second approach, stereotactic CT scanning is performed using a fiducial system when acquiring the images. Non-stereotactic MRI scans are also obtained (see Fig. 6.5). The MRI scans are then mapped into stereotactic space by registration of the MRI dataset to the CT dataset. In most cases, rigid registration techniques are used. Typically, the clinician visually inspects the results of the registration to verify the quality of the fit. An advantage of this second approach is that it allows the MRI scan to be obtained prior to the placement of any stereotactic ring. Consequently, the clinician can exam the actual scan that will be used for scanning prior to deciding that radiosurgery is the appropriate mode for treatment.
Contouring Structures
After the images have been imported into the planning system and the fiducial marks have been registered, the target to be treated is then identified. To aid target delineation, fusion of images from different imaging modalities is possible with many SRS planning systems. While some planning systems may not require the users to outline the target and critical structures, contouring is required if plan evaluation tools such dose-volume histograms (DVHs) are to be used. Contouring is also required by systems that employ inverse planning.
The patient’s external contour can either be manually contoured or identified automatically by the planning system based on grayscale information from the images. For Gamma Knife planning, the skin contour or scalp of the patient’s head is measured with a skull-scaling device (see Fig. 6.6). The location of the surface is important for computing the penetration depth for each beam during the dose calculation. The skull-scaling device used for Gamma Knife planning makes it possible to know the penetration depth for each photon beam even if the entire skull has not been imaged.
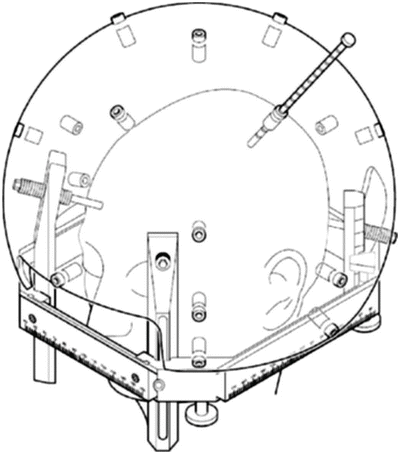
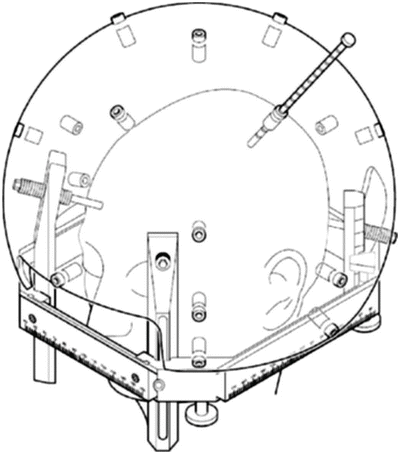
Fig. 6.6
Skull-scaling device used with the Gamma Knife to provide mapping of the skull (Photo courtesy of Elekta)
Defining the Prescription
The dosimetric characteristics of the treatment plan vary from one delivery approach to another. With the Gamma Knife and linac-based SRS using cones, the use of multiple isocenters means that multiple spherical isodose distributions have to be packed into the target volume. Overlaps of such high-dose spheres are inevitable. As a result, the dose is highly nonuniform in the target. The isodose surface that encloses the target (which is often taken as the prescription dose) is typically 50 % of the maximum dose in the target.
For linac-based SRS using an MLC, the target dose is highly uniform when a single isocenter is used. The isodose line that encompasses the target can be greater than 80 % of the maximum dose in the target. In SRS, the prescription dose is normally set to the dose level that conforms to the target, or the minimum target dose. As compared with fractionated radiation treatments, radiosurgery treatment plans have significantly less normal tissue included in the prescription isodose volume. For this reason, the typical restrictions on dose uniformity (a foundation of fractionated radiation therapy) do not apply in radiosurgery cases. The radiosurgeon is more concerned with the dose to normal tissue outside the target volume. Consequently, the goal in treatment planning is to achieve maximum normal tissue sparing rather than maximum target dose uniformity.
The radiosurgeon can minimize the dose to normal tissue by designing a plan where the isodose that just covers the target surface is along the steepest portion of the dose gradient. It can be shown that for single-isocenter plans, this places the optimal dose prescription at the 80 % isodose surface [16]. This is because the dose gradient falls off most quickly, between the 80 and 40 % isodose surfaces. Therefore, prescribing to the 80 % isodose line minimizes the integral dose to all normal tissues, the tissues outside the target volume. For multiple isocenter plans, this optimal surface shifts to the 70 % isodose surface and at time can be extended to isodose surfaces as low as the 50 % dose surface, as is often the case in Gamma Knife plans.
Designing the Treatment Plan
For optimal planning, the neurosurgeon, radiation oncologist, and physicist or dosimetrist should perform the treatment planning as a team and bring all aspects of expertise to bear on the problem. After the images have been imported and registered and contouring of structures has been completed, one can proceed with the task of formulating a treatment plan that meets the dosimetric requirements specified by the physician. The planning steps that are followed differ from one delivery technique to the next. For the Gamma Knife and for linac-based SRS with circular collimators, the task of planning is to find a set of isocenter locations, the size of the collimators to use for each location, and the weights of the isocenters. Multiple isocenters are commonly used in a Gamma Knife treatment plan due to the limited number of collimator sizes to choose from and the relative efficiency with which each isocenter can be delivered [17–24]. For linac-based SRS with circular collimators, the planner strives to minimize the number of isocenters in a plan to compensate for the relative inefficiency of the beam delivery [25, 26]. Linac systems, however, have the advantage of a much wider range of collimators usually extending from 5 to 50 mm in diameter.
For linac-based SRS with MLCs, only one isocenter is used [25, 27–37], and the planning methods are similar to those used for external beam radiation therapy. The selection of beam directions and field shapes is aided by the use of tools such as beam’s-eye-view (BEV) visualization and digitally reconstructed radiographs (DRRs).
Dose Calculation
A variety of dose calculation techniques have been employed for SRS treatment planning [38–48]. Simple empirical dose calculation methods were employed with many of the earlier SRS planning systems [17]. For Gamma Knife radiosurgery, a standard set of beam data is included with the system, and the user verifies the dose profiles of an individual beams for each of the focusing helmets. The use of a standard dataset is possible because all Gamma Knife systems use the same design with only slight variations in the single beam dosimetry. The dose distribution in the patient is calculated by adding the dose distributions for all 192 beams [20]. For linac-based SRS with circular collimators, the tissue-maximum ratios (TMRs) and dose profiles can be measured for each collimator size as a function of depths. For a given arc, the mean TMR for all beam directions is calculated and the total dose contributed by the arc can be calculated. Dose distributions can be computed by approximating an arc as a series fixed beams and summing the dose distributions from all of the beams.
For intracranial SRS, heterogeneity corrections are generally not applied in the dose calculation. Corrections to account for variations in the attenuating properties of tissue, bone, and air are not needed because of the simple geometry that is employed [44, 49]. The magnitude of the resulting error can be estimated by assuming that the average beam passes through 5 mm of skull with an average density of 1.2 g/cc. Assuming an attenuation of 4 %/cm for a 6 MV beam, the maximum error in absolute TMR that would result from ignoring heterogeneity corrections is less than 1 %. However, when SRS is extended to extracranial applications, traditional empirical dose calculation methods are no longer adequate for accurate dose calculations due to the presence of bone and air. Therefore, many treatment-planning systems designed for planning extracranial SRS employ 3D pencil beam dose calculation methods.
Forward Versus Inverse Planning
Forward planning is the most common planning approach for SRS. With forward planning, the treatment plan is developed through an iterative trial and error approach. From one iteration to the next, the planner attempts to determine a set of parameters (such as beam angle, beam weights) that give an acceptable plan. The iterative process is usually stopped when the planner is no longer able to make noticeable improvements in the plan quality.
With inverse treatment planning, the user begins by outlining the target and any sensitive structures. A series of treatment goals are then defined, and an optimization algorithm determines the plan parameters that provide a plan that best satisfies these goals. The quality of the plan is scored using an objective function and constraints. An objective function reduces an entire treatment plan into a single numerical value. The job of the optimizer is to either minimize or maximize the value of the objective function. Typically, an optimization will also include one or more constraints. A constraint is a condition that must be satisfied in order for a solution to be considered feasible. The most basic constraint in any radiotherapy optimization is that the beam weights must be nonnegative.
As compared with forward planning, inverse planning provides two potential advantages. First, the time required for planning can be reduced because much of the trial and error is removed. Secondly, inverse planning should lead to improved plan quality due to the ability of the optimizer to consider thousands of plan configurations in selecting the optimal plan. Unfortunately, the quality of inverse planning tools for SRS varies significantly from one delivery technique to the next and one planning system to the next. Users of some systems will find that an experienced planner using forward planning techniques produces the highest quality plans.
Evaluation of Plan Quality
The most common tool for evaluating plan quality is the visualization of isodose curves superimposed on the patient’s anatomical images. Figure 6.7a shows a typical isodose plot for a Gamma Knife patient. In this case, the isodose curves are plotted as a percentage of the maximum target dose. Isodose curves can also be plotted as a percentage of the prescribed dose or as absolute dose lines. When evaluating plan quality, it is common practice to visualize isodose curves in the axial, sagittal, and coronal planes. Some systems can also display three-dimensional dose clouds that make it possible to quickly assess the quality of the dose coverage.
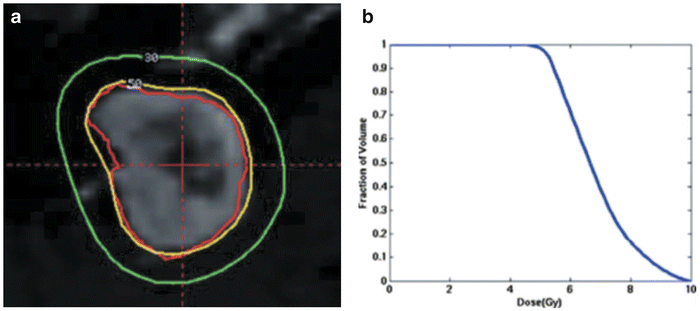
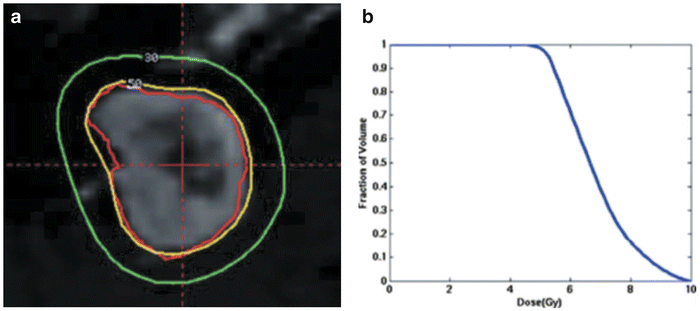
Fig. 6.7
(a) A Gamma Knife isodose plot with the target outlined in red, the 50 % isodose line in yellow, and the 30 % isodose line in green. (b) Dose-volume histogram for target in this case
DVHs also serve as an important tool for analyzing plan quality. A DVH plot from a Gamma Knife patient is shown in Fig. 6.7b. For each structure, the DVH plots the fraction of the volume covered by each dose level. Both the dose and the volume can be expressed as either absolute or relative values. DVHs are particularly useful because they reduce a three-dimensional treatment plan into an easy to read two-dimensional plot. The comparison of multiple plans is also simplified by overlying DVHs on the same plot. The disadvantage of DVHs is the lack of spatial information. A DVH will indicate the presence of hot or cold spots, but it does not provide specify where in the structure of interest this underdosage or underdosage is located. Consequently, slice-by-slice evaluation of a plan is critical in making a final decision regarding a treatment plan.
Additional parameters have been defined to score both dose conformity and target coverage for SRS treatment plans [50–58]. The most commonly used parameter for scoring dose conformity is the conformity index defined in the radiosurgery quality assurance guidelines of the Radiation Therapy Oncology Group (RTOG) [52]. The RTOG defined the conformity index as the volume of the prescription isodose surface divided by the target volume. For a perfectly conformal plan where the prescription isodose line exactly matches the target volume, the conformity index would equal 1. A case is considered to be per protocol if this ratio falls between 1.0 and 2.0. A shortcoming of this index is that it does not consider the degree of overlap between the prescription isodose curve and the target. A plan with a complete geometric miss of the target could still give a perfect conformity index. As an alternative, Lomax and Scheib have suggested a conformity index defined as “the ratio of the volume within the target irradiated to at least the prescription isodose over the total volume enclosed by the prescription isodose” [55]. Consequently, this value ranges from 0 (no conformity) to 1.0 (for perfect conformation, where the prescription isodose is identical to the target volume). As a planning goal, Lomax and Scheib suggest a conformity index of 0.6 or higher.
The RTOG has also defined a homogeneity index that is equal to the maximum dose in the treatment volume divided by the prescription dose. A case is considered per protocol if this ratio is less than or equal to 2.0. In terms of target coverage, the RTOG considers a case to be per protocol if the isodose line equal to 90 % of the prescribed dose completely encompasses the target. Lomax and Scheib suggest an alternative volumetric definition of coverage where target coverage is defined as the percentage of the target volume covered by the prescription [55].
Gamma Knife
Gamma Knife Unit
The first Gamma Knife was built in 1967 under the direction of Lars Leksell of the Karolinska Institute in Stockholm, Sweden. Currently, there are over 300 units worldwide with approximately 60,000 patients treated annually [59]. Over the years, there have been a number of advances and updates to the Gamma Knife. Two models of the Gamma Knife are currently offered by Elekta, the 4C (introduced in 2004) and the Perfexion (introduced in 2006).
Gamma Knife 4C
Inside of the shielded treatment unit of the Gamma Knife 4C (Fig. 6.8), the beams from 201 radioactive sources are focused so that they intersect at a single location. The result is an elliptical region of high dose with a rapid falloff in dose outside of the boundaries of the ellipse. Each exposure to an elliptical region of high dose is referred to as a “shot” of radiation.
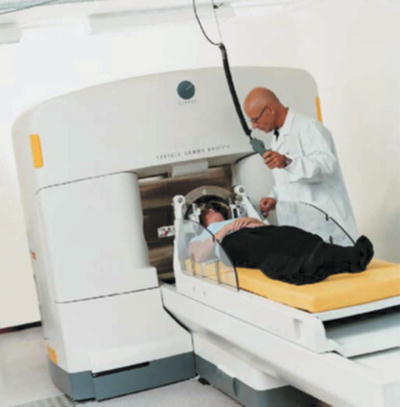
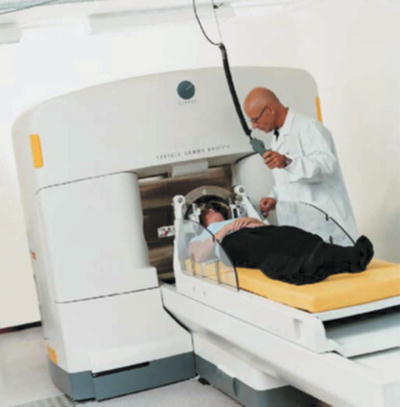
Fig. 6.8
A patient positioned for treatment on a Gamma Knife model 4C (Photo courtesy of Elekta)
For each exposure, the focusing helmet dictates the size of the high-dose region. A focusing helmet incorporates a separate collimator for each of the 201 Co-60 sources (see Fig. 6.9a). The four focusing helmets provided with the Gamma Knife can be used to produce a shot of radiation that is 4, 8, 14, or 18 mm in diameter (see Fig. 6.9b). Within each helmet, individual collimators may be plugged to provide further shaping of the dose distribution. The benefits of plugging are illustrated in Fig. 6.9.
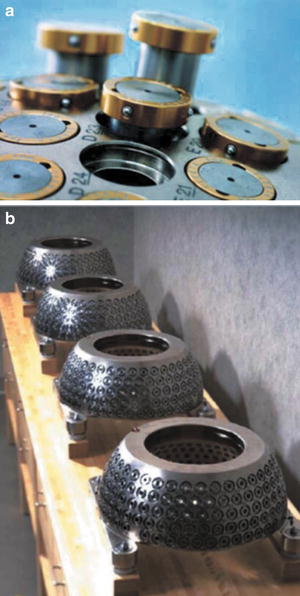
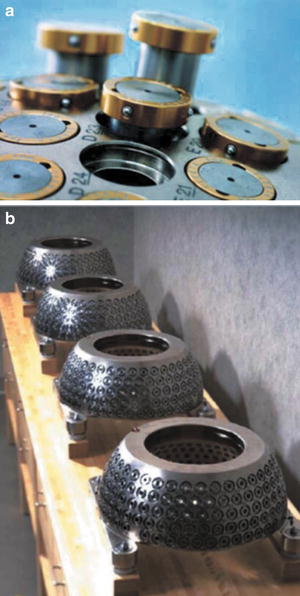
Fig. 6.9
The four focusing helmets dictate the size of each shot of radiation (Photo courtesy of Elekta)
Figure 6.10a shows the isodose curve for a single 4 mm shot for a trigeminal neuralgia patient. In Fig. 6.10b, a plugging pattern has been used to lower the dose to the brainstem. The plugging pattern is shown in Fig. 6.10c.
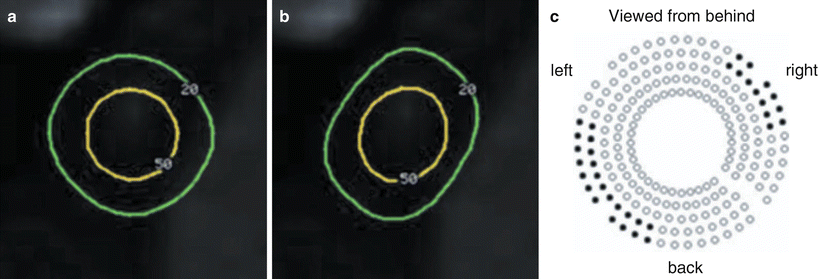
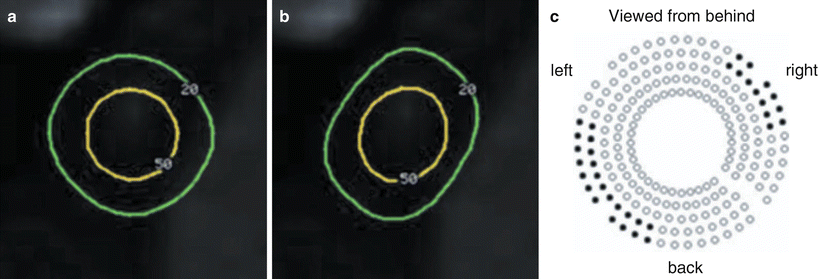
Fig. 6.10
The isodose curves for a trigeminal neuralgia patient before (a) and after (b) plugging. (c) The plugging pattern. 35 of the 201 sources have been blocked (blocked collimators are shown in black)
After the imaging is complete, the patient images are loaded into the Gamma Knife’s treatment-planning system (GammaPlan). The images are registered with respect to the fiducial marks, and the treatment volume is outlined by a physician.
At the time of treatment, the patient lies on the couch of the treatment unit, and the appropriate focusing helmet is affixed to the table. The patient’s stereotactic head frame is then attached to the focusing helmet, and the x, y, and z coordinates are adjusted to place the center of the planned shot of radiation at the focal point of the 201 beams of radiation. After, the personnel leave the room, the exposure time is programmed, the door of the shielding unit is opened, and the couch is advanced into the high radiation field. Next, the treatment couch is docked, and the shot of radiation is delivered. After the exposure is complete, the couch retracts and the door closes. If more than one shot of radiation is to be delivered, the process is repeated with the appropriate focusing helmet and x, y, and z positioning. The model 4C Gamma Knife provides an automated positioning system in which the shot positions and exposure times are directly transferred to a record-and-verify system, and the machine sets the coordinates in an automated fashion.
The treatment planning for the Gamma Knife 4C is relatively straightforward for small spherical targets. For example, Fig. 6.11 shows a case where the treatment volume is relatively spherical and approximately 6 mm in diameter. An 8 mm shot of radiation was placed so that it covers the entire tumor volume.
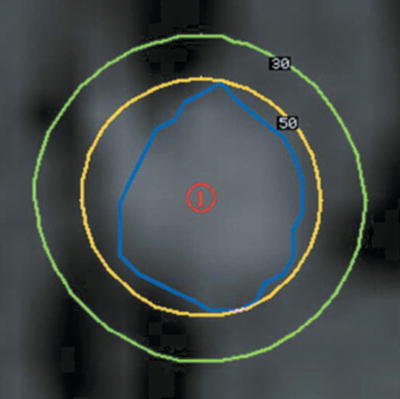
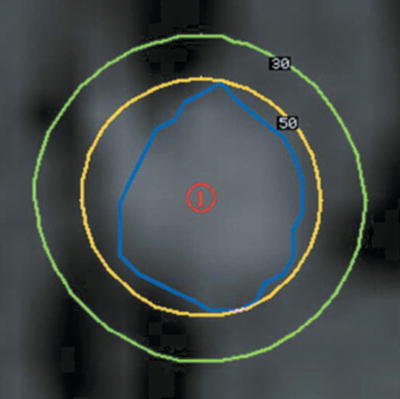
Fig. 6.11
A single shot treatment plan for a Gamma Knife treatment. The target outlined in blue, the 50 % isodose line in yellow, and the 30 % isodose line in green
The treatment-planning process becomes more complex when the tumor volume is large or irregularly shaped. These cases typically require several shots of radiation. Through an iterative process, the planner must determine the number of shots of radiation that are required along with the size, the location, and the weight that should be assigned to each.
In Fig. 6.12, a simple 2D bean-shaped target is used to illustrate the general planning procedure [22]. Each frame illustrates the dosimetric impact of an added shot of radiation. In this case, five shots of radiation provide a conformal treatment plan. Note that three different shot sizes were used in creating this plan.
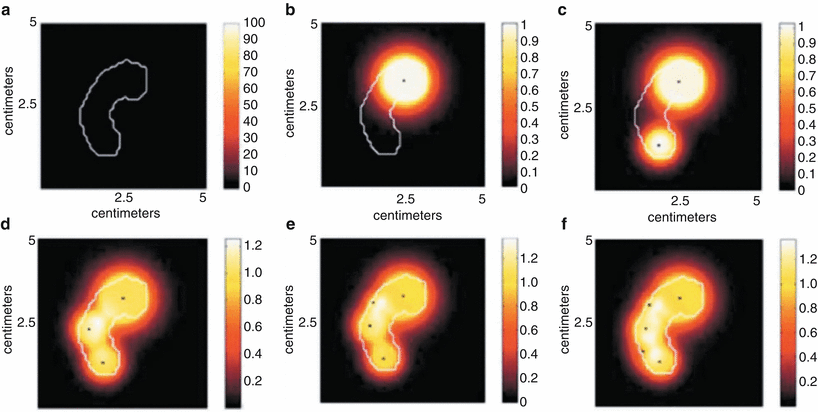
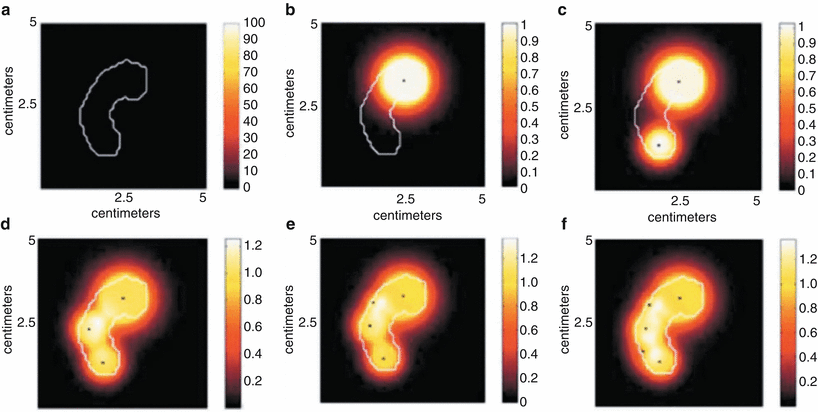
Fig. 6.12
(a) A bean-shaped target. (b–f) The process of adding shots to create a treatment plan is illustrated
During the process of treatment planning, the plan quality is evaluated through the use of isodose plots and DVHs. In the GammaPlan system (the Gamma Knife specific treatment-planning system), users typically normalize to the maximum dose and seek to cover the target with the 50 % isodose line.
Gamma Knife Perfexion
In 2006, Elekta introduced the Gamma Knife Perfexion which includes a significant redesign of the Gamma Knife. The most critical change in the Perfexion is the new collimator system. The new system replaces the multi-helmet collimator setup with a single integrated permanent collimator system that incorporates openings for 4, 8, and 16 mm treatment beams.
The collimator is partitioned into eight independently moveable sectors each delivering 24 beams of radiation meaning there is a total of 192 Co-60 sources. The beam size can be changed dynamically by sector, and individual sectors can be blocked to provide further shaping of each shot of radiation. For each shot, an ellipsoidal shot of radiation can be delivered by utilizing the same sized beams from each of the eight sectors. More sophisticated shaping of the dose distribution can be obtained by mixing beams of various sizes and/or blocking individual sectors. Unlike with the model 4C where it is necessary to manually block individual beams to shape the dose distribution, the Perfexion makes it possible to simply move individual sectors into the off position to block the beams that pass directly through a particular critical structure.
In addition to the updated collimator design, the Perfexion introduces a number of other changes to the Gamma Knife. One change is in the positioning system design. The Perfexion positions the patient by moving the couch rather than moving patient’s head. Rapid transition times are achieved moving from one shot of radiation to the next because there is no need to change focusing helmets and the beams are simply moved into the off position during the transition. This ability to quickly switch from one shot of radiation to the next facilitates the treatment of larger tumors as well as patients with multiple brain metastases.
The Perfexion provides improved patient comfort due the increased space inside the collimator body. The larger collimator size also eliminates the need for eccentric frame positioning, and makes it possible to treat most peripheral lesions. The Perfexion is also the first Gamma Knife model that offers a stereotactic frame with a vacuum-assisted bite block (the Extend system) that makes it possible to deliver frameless fractionated treatments.
Linac-Based SRS with Circular Cones
Initial developments in the use of linear accelerator-based SRS techniques were centered on the use of multiple converging arc treatments delivered with circular collimators. For each treatment plan, the user must select the number of arcs, the angular arrangement of each arc, the weight assigned to each arc, and the number of isocenters. When one isocenter is used, the high isodose levels at the isocenter are nearly spherical. A variety of arc arrangements have been reported [26, 60]. As compared with the Gamma Knife, a greater number collimator sizes is available with circular collimators. By adjusting the weightings of the arcs or through an asymmetric arc arrangement, ellipsoidal instead of spherical isodose distributions can be created.
For tumors that are small and convex in shape, it is often possible to treat with only one isocenter. When one isocenter is used, the dose uniformity in the target is high and the dose can be prescribed to a high isodose level. When the target volume is large or it deviates from a sphere or an ellipsoid, multiple isocenters are typically required to achieve adequate target dose conformity. The spherical high-dose regions must overlap in order to avoid leaving a cold spot in the target. Consequently, one must accept a decreased target dose uniformity as compared to plans using a single isocenter. To date, no negative clinical consequences have been reported as a result of the lack of dose uniformity seen in Gamma Knife and linac-based circular collimator treatments.
When multiple isocenters are used, the treatment-planning problem is similar to sphere packing problem of the Gamma Knife [61], where spherical dose distributions are packed together to achieve a composite dose distribution conforming to the target volume. Because the setup and delivery time per isocenter is typically longer for linac-based radiosurgery as compared with Gamma Knife-based radiosurgery, it is generally desirable to deliver a limited number of isocenters. However, the greater selection of collimator sizes and the availability of larger collimator sizes as compared with the Gamma Knife reduce the need for the use of a large number of isocenters. Overall, the Gamma Knife centers and linac-based SRS centers have reported similar cure rates and complication levels.
Linac-Based SRS with Multileaf Collimators
An MLC is a field-shaping device that uses movable leaves made out of a highly attenuating material such as tungsten in order to generate arbitrary field shapes (see Fig. 6.13). The first MLCs designed for routine external beam delivery typically had leaf widths that projected to 1 cm at isocenter. These MLCs lacked the geometric precision for shaping small irregularly shaped fields such as those in commonly encountered in SRS.
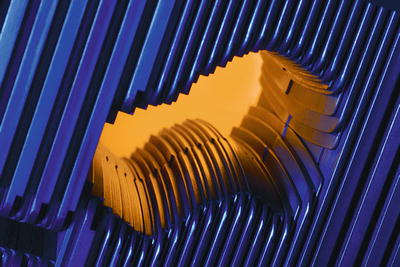
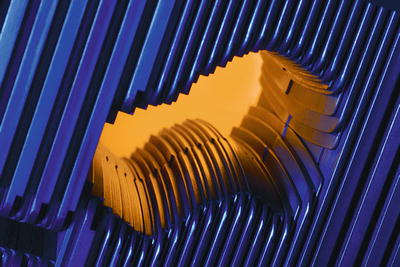
Fig. 6.13
A photo of Varian’s high definition multileaf collimator (HD-MLC) (Varian Medical System, Inc. All rights reserved.)
Interest in the use of MLCs for radiosurgery increased when vendors introduced micromultileaf collimators (mMLCs), a type of MLC where each leaf projects to a width of between 2 and 5 mm at isocenter. mMLCs are suitable for SRS applications, because they are capable of shaping small irregular fields with acceptable geometric error. Typical mMLCs have between 20 and 80 leaves, arranged in pairs. The maximum field size of mMLCs generally varies from 8 to 20 cm, much greater than those available with traditional circular collimators. As a result, extracranial SRS and stereotactic body radiation therapy (SBRT) can be delivered.
Additionally, vendors now offer MLCs designed for both conventional treatments and radiosurgery. For example, Elekta’s Agility MLC has 160 leaves of projected width 0.5 cm at isocenter and offers field sizes up to 40 × 40 cm. Varian offers their HD 120™ MLC, a 120 leaf MLC which can deliver field sizes up to 22 × 40 cm with 2.5 mm leaves (projected at isocenter) across the central 8 cm of the field.
Due to their ease of use and wide range of possible applications, the advent of MLCs has led to a decline in popularity of SRS delivery using circular collimators.
As compared with Gamma Knife and linac-based SRS with circular collimators, the use of an MLC and a single isocenter can lead to plans that are less conformal with a less steep dose gradient at the target edge. The decreased dose gradient is in part the result of the use of larger beams and fewer unique beam paths. As mentioned previously, a routine Gamma Knife plan will involve as many as 201 beams and a typical plan for a linac-based cone-beam treatment would include five arcs each covering approximately 100° of gantry rotation. By contrast, a typical micromultileaf plan may have as few as six beams.
Target size is a second issue that impacts one’s ability to create a conformal treatment plan using an MLC. For small targets, such as in the treatment of trigeminal neuralgia, high precision in target localization and positioning is required. MLCs may not be suitable for target sizes significantly less than one centimeter due to the undulating field edges caused by the finite leaf width. For larger targets, MLC can provide efficient beam delivery and dose uniformity due to the use of a single isocenter [50]. However, the dose to surrounding structures increases as the size of the target increases [50]. As a result, the dose to normal structures may exceed the acceptable limits if one attempts to treat a large tumor with a single fraction.
Generally multileaf collimators (including mMLCs) can be used for four delivery approaches: (1) fixed-field conformal delivery; (2) dynamic conformal arc delivery; (3) fixed-field intensity-modulated radiation therapy (IMRT); and (4) volumetric modulated arc therapy (VMAT).
Fixed Fields: 3D Conformal Delivery
With MLCs, three-dimensional (3D) conformal therapy treatment-planning techniques can be applied to SRS planning. If there are a sufficient number of MLC-shaped beams, the dose distribution quality can rival that obtained using with multiple arcs using circular collimators and multiple isocenters. This is especially true when the targets are larger and non-spherical in shape. Because only one isocenter is needed, the dose uniformity created with MLCs is generally better than that achieved with the use of circular collimators and multiple isocenters. With the exception of the need for stereotactic reconstruction from stereotactic frames and markers, the planning method for SRS essentially matches that for 3D conformal therapy. Each non-coplanar field is shaped based on the BEV of the target with additional margins to account for the width of the beam penumbra, which is wider than the penumbra from circular collimators and increases with leaf width.
The use of fixed fields delivered with an MLC is best suited for convex target shapes. With fixed fields, there is only a limited ability spare normal tissues in the center of a concave volume.
Dynamic Conformal Arcs
In dynamic conformal arc delivery, the shape of the field changes continuously to conform to the BEV of the target during arced beam delivery. Dynamic conformal arc delivery combines the dosimetric advantages of arcs (reduced hot-streaks of dose through the patient) with the dose conformity that is possible with MLC beam shaping. Additionally, this approach only requires a single isocenter. With the use of three or four non-coplanar arcs, a uniform high-dose volume can be created that conforms to the target.
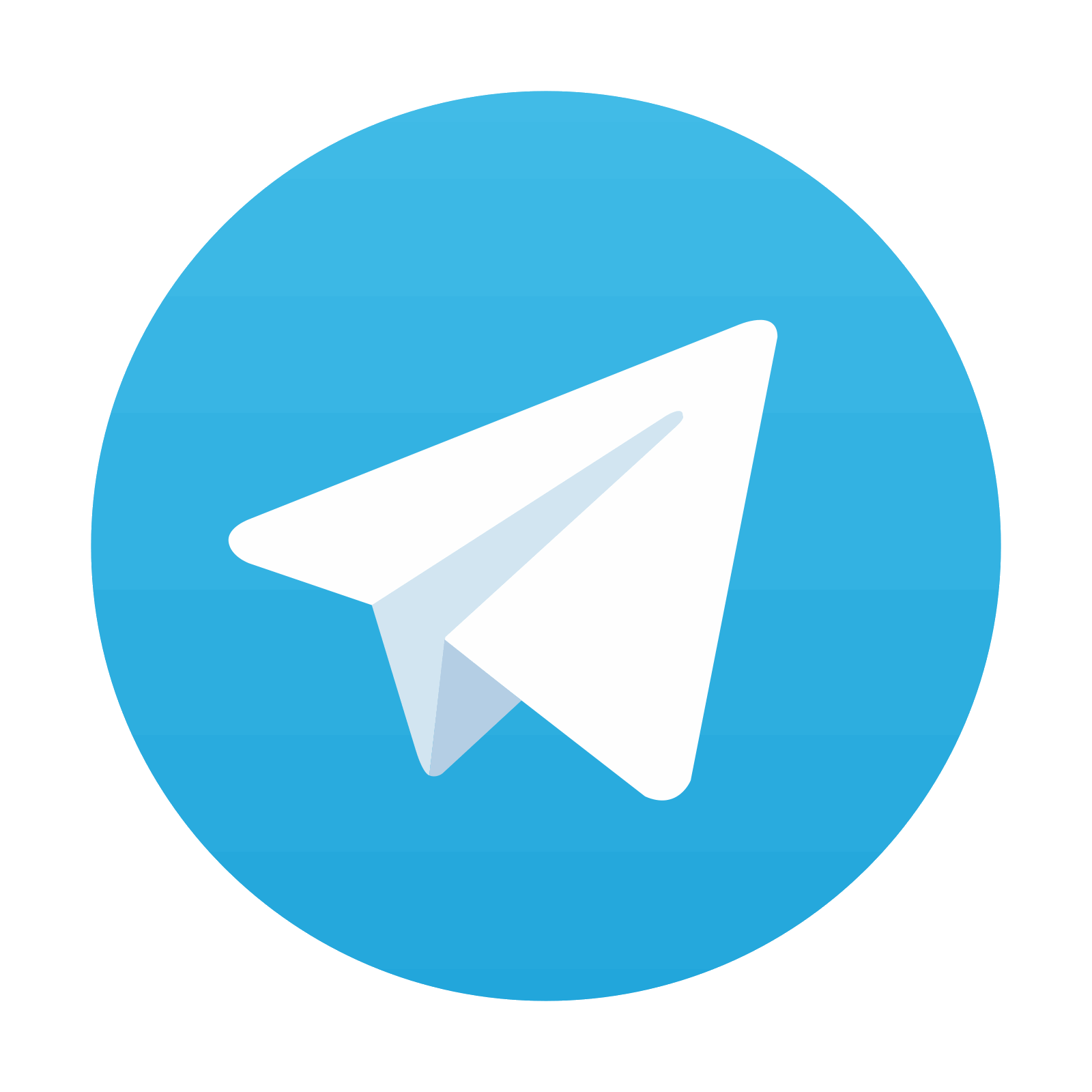
Stay updated, free articles. Join our Telegram channel
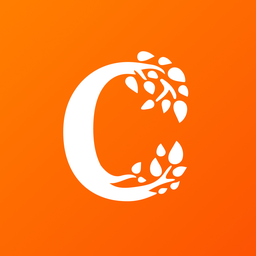
Full access? Get Clinical Tree
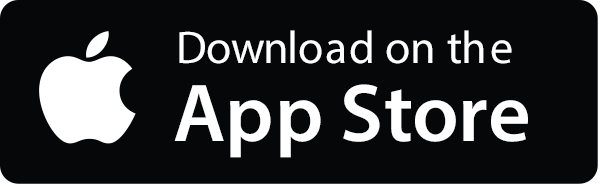
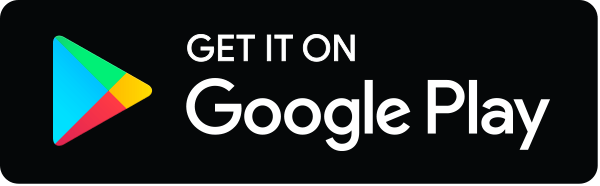