1
Radiography, Computed Tomography, Magnetic Resonance Imaging, and Ultrasonography: Principles and Indications
Vincent A. Magnotta • Wilbur L. Smith • William E. Erkonen
Chapter Outline
Computed Radiography (Digital Radiography)
Multislice/Dynamic Computed Tomography
Dual-Source Computed Tomography
Magnetic Resonance Angiography
Functional Magnetic Resonance Imaging
Functional Cardiac Magnetic Resonance Imaging
Diffusion-Weighted Imaging Magnetic Resonance
Susceptibility-Weighted Magnetic Resonance Imaging
Magnetic Resonance Spectroscopy
Few of us take the time to study, let alone enjoy, the physics of the technology that we use in our everyday lives. Almost everybody drives an automobile, for instance, but only a few of us have working knowledge about what goes on under our car hoods. The medical technology that produces imaging studies is often met with a similar reception: We all want to drive the car, so to speak, but we do not necessarily want to understand the principles underlying the computed tomograms or magnetic resonance (MR) images that we study. Yet, a basic understanding of imaging modalities is extremely important, because you will most likely be reviewing images throughout your professional career and the results of these consultations will affect your making a clinical decision. The interpretation of imaging studies is to a considerable degree dependent on understanding how the images are produced. One does not necessarily have to be a mechanic to be a skilled driver, but you do need to know when to put fuel in the car. Similarly, reaching a basic understanding of how imaging studies are produced is a necessary first step to critically viewing the images themselves. This chapter is designed to demonstrate the elementary physics of radiologic diagnostic imaging.
RADIOGRAPHY
Radiographs are the most common imaging consultations requested by clinicians. So let us set off on the right foot by referring to radiologic images as radiographs, images, or films, but not x-rays. After all, x-rays are electromagnetic waves produced in an x-ray tube. It is acceptable for a layperson to refer to a radiograph as an x-ray, but the knowledgeable clinician and healthcare worker should avoid the term. Your usage of appropriate terminology demonstrates your savoir-faire (the ability to say and do the right thing) to your colleagues and patients.
Whenever possible, radiographs are accomplished in the radiology department. The number of views obtained during a standard or routine study depends on the anatomic site being imaged. The common radiographic views are named according to the direction of the x-ray beam and referred to as posteroanterior (PA), anteroposterior (AP), oblique, and lateral views.
The chest will be used to illustrate these basic radiographic terms, but this terminology applies to almost all anatomic sites. PA indicates that the central x-ray beam travels from posterior to anterior or back to front as it traverses the chest or any other anatomic site (Fig. 1.1). Lateral indicates that the x-ray beam travels through the patient from side to side (Fig. 1.2). When the patient is unable to cooperate for these routine views, a single AP upright or supine view is obtained. AP means that the x-ray beam passes through the chest or other anatomic site from anterior to posterior or front to back (Fig. 1.3). PA and AP radiographs have similar appearances but subtle difference in magnification of structures, particularly the heart. When the patient cannot tolerate a transfer to the radiology facility, a portable study is obtained, which means that a portable x-ray machine is brought to the patient wherever he or she is located. AP is the standard portable technique with the patient sitting or supine (Fig. 1.4). Portable radiographic equipment generates less powerful x-ray beams than fixed units and therefore, the prevalence of suboptimal images is greater.
FIGURE 1.1. A posteroanterior chest radiograph. The patient’s chest is pressed against the cassette with hands on the hips. The x-ray beam emanating from the x-ray tube passes through the patient’s chest in a posterior-to-anterior or back-to-front direction. The x-rays that pass completely through the patient eventually strike the radiographic film and screens inside the radiographic cassette.
Radiographs have traditionally been described in terms of shades of black, white, and gray. What causes a structure to appear black, white, or gray on a radiograph? Actually, it is the density of the object being imaged that determines how much of the x-ray beam will be absorbed or attenuated (Fig. 1.5). In other words, as the density of an object increases, fewer x-rays pass through it. It is the variable density of structures that results in the four basic radiographic classifications: Air (black), fat (black), water (gray), and metal or bone (white; Table 1.1). For example, the lungs primarily consist of low-density air, which absorbs very little of the x-ray beam. Thus, air allows a large amount of the x-ray beam to strike or expose the radiographic film. As a result, air in the lungs will appear black on a radiograph. Similarly, fat has a low density, but its density is slightly greater than that of air. Fat will appear black on a radiograph but slightly less black than air. High-density objects such as bones, teeth, calcium deposits in tumors, metallic foreign bodies, right and left lead film markers, and intravascularly injected contrast media absorb all or nearly all of the x-ray beam. As a result, the radiographic film receives little or no x-ray exposure, and these dense structures appear white. Muscles, organs (heart, liver, spleen), and other soft tissues appear as shades of gray, and the shades of gray range somewhere between white and black depending on the structure’s density. These shades of gray are referred to as water density.
FIGURE 1.2. A lateral chest radiograph. The x-ray beam passes through the patient’s chest from side to side. The x-rays that pass completely through the patient eventually strike the radiographic film and screens. Note that the patient’s arms are positioned as not to project over the chest.
FIGURE 1.3. An anteroposterior chest radiograph. The x-ray beam passes through the patient’s chest in an anterior-to-posterior or front-to-back direction. Note that the patient’s hands are on the hips.
FIGURE 1.4. An anteroposterior portable chest radiograph with the patient either sitting (A) or supine (B). The x-ray beam passes through the patient’s chest in an anterior-to-posterior direction. The x-ray machine has wheels and this allows it to be used wherever needed throughout the hospital.
FIGURE 1.5. A: The level in the distal thigh through which the x-ray beam is passing in (B). B: Cross-section of the distal thigh at the level indicated in (A). Notice that when the x-ray beam passes through air, the result is a black area on the radiograph. When the x-ray beam strikes bone, the result is a white area on the radiograph. If the x-ray beam passes through soft tissues, the result is a gray appearance on the film.
Table 1.1
In the “old days” when films were widely employed as an image storage/display medium, radiographic screens are positioned on both sides of a sheet of film inside the lighttight cassette or film holder (Fig. 1.6A). The chemical structure of the screens causes them to emit light flashes or to fluoresce when struck by x-rays (Fig. 1.6B). Actually, it is the fluoresced light from the screens on both sides of the film that accounts for the major exposure of the radiographic film. The direct incident x-rays striking the radiographic film account for only a small proportion of the film exposure. The use of screens decreases the amount of radiation required to produce a radiograph, and this in turn decreases the patient’s exposure to radiation. It is important to remember that radiographic films, photographic films, and the currently used phosphor plates for digital radiography (DR) all respond in a similar manner to light and x-rays. While film recording is going the way of the dodo, this principal remains valid.
Computed Radiography (Digital Radiography)
In conventional radiography, the radiographic image is recorded on film that goes through chemical processing for development. Computed radiography (CR) or digital radiography (DR) is the process of producing a digital radiographic image. Instead of film, a special phosphor plate is exposed to the x-ray beam. The image information is obtained by scanning the phosphor plate with a laser beam that causes light to be released from the phosphor plate. The intensity of the emitted light depends on the local radiation exposure. This emitted light is intensified by a photomultiplier tube and is subsequently converted into an electron stream. The electron stream is digitized, and the digital data are converted into an image by computer. The resulting image can be viewed on a monitor or transferred to a radiographic film. The beauty of this system is that the digital image can be transferred via networks to multiple sites in or out of the hospital, and the digital images are easily stored in a computer or on a server. For example, a digital chest radiograph obtained in an intensive care unit can be transmitted to the radiology department for consultation and interpretation in a matter of seconds. Then the radiologist can send this image via a network back to the intensive care unit or to the referring physician’s office and this digital information would be stored in a computer (server) for future recall. This technology is used routinely in the practice of medicine to share images between the radiologist and referring physicians.
FIGURE 1.6. A: An open radiographic cassette containing one sheet of radiographic film and two intensifying screens. A radiographic screen is positioned on each side of the film, and the screens emit a light flash (fluoresce) when struck by an x-ray. Also, some x-rays directly strike the radiographic film. This combination of light flashes from the screens and x-rays directly striking the film causes the radiographic film to be exposed. This is similar to photographic film. B: Cross-sectional illustration of a radiographic cassette. Note the lead foil in the back of the cassette that is designed to stop any x-rays that have penetrated the full thickness of the cassette. The curved arrows represent light flashes that are created when x-rays strike the screens.
Contrast Media
Radiographic contrast media usually refer to the use of intravascular pharmaceuticals to differentiate between normal and abnormal tissues, to define vascular anatomy, and to improve visualization of some organs. These high-density pharmaceuticals in conventional radiology depend upon chemically bound molecules of iodine that cause varying degrees of x-ray absorption. Soft tissues such as muscles, blood vessels, organs, and some diseased tissues often appear similar on a radiograph. Usually, when contrast agents are injected intravascularly to tell the difference between normal and abnormal tissues there is a difference in the uptake of the contrast media in the various tissues. Thus, the more the uptake of contrast media in a tissue, the whiter it appears, and this is called enhancement.
It is this enhancement or contrast that enables the viewer to detect subtle differences between normal and abnormal soft tissues and between an organ and the surrounding tissues. Also, it beautifully demonstrates arteries and veins.
The use of iodinated high-osmolar contrast agents for radiographic studies through the years has led to complications due to this high-osmolar load especially in infants and in individuals with compromised renal function. With high-osmolar contrast agents, approximately 7% of the people developed reactions consisting of vomiting, pain at the injection site, respiratory symptoms, urticaria, and generalized burning sensation. However, a major advance occurred in the 1990s with the widespread adoption of low-osmolar contrast agents (LOCAs) that substantially reduced the risk of osmolar reactions. LOCAs improved the comfort of administration and decreased the frequency of annoying and sometimes life-threatening reactions. LOCAs did not completely eliminate the incidence of serious contrast reaction and nephropathy. If a patient has had a prior reaction, one should consult with one’s radiologist to weigh the benefit versus the risk and possible alternative imaging considered especially in patients with diabetes, vascular disease, or renal dysfunction.
There are many uses for iodinated compounds in radiographic examinations such as in angiography, myelography, arthrography, and computed tomography (CT). Angiography is merely the injection of an iodinated contrast media directly into a vein or artery via a needle and/or catheter (see Chapter 11). Arthrography is the injection of contrast media and/or air into a joint. Air may be used alone or in combination with these compounds to improve contrast. It has been used to image multiple joints such as rotator cuff injuries of the shoulder and to assess meniscus injuries in the knee. Since the advent of CT and magnetic resonance imaging (MRI), the arthrogram has become less important. Myelography is the placement of contrast media in the spinal subarachnoid space, usually via a lumbar puncture. This procedure is useful for diagnosing diseases in and around the spinal canal and cord. Because of the advent of the less invasive CT and MRI modalities, the use of myelogram studies has been decreasing.
Another type of contrast media is used for the gastrointestinal (GI) tract. A heavy metal-based compound (usually barium) defines the mucosal pattern very well. To accomplish a GI contrast examination, the barium sulfate suspension is introduced into the GI tract by oral ingestion (upper GI series) or through an intestinal tube (small bowel series) or as an enema (barium enema). When air along with the barium is introduced into the GI tract, the result is called a double-contrast study. Barium studies are safer, better tolerated by patients, and relatively inexpensive compared with the more invasive GI endoscopic studies. Barium studies can be effective in diagnosing a wide variety of GI pathology, as they are quite sensitive and specific. With the widespread use of CT to study GI pathology, both barium- and iodine-based contrast agents have been utilized. Owing to the contrast sensitivity of CT, a much lower concentration (not volume) of barium or iodine is employed for bowel visualization.
When the integrity of the GI tract is in question, there exists a potential for catastrophic extravasation of the barium into the mediastinum and peritoneum. In these situations, barium studies are contraindicated and a water-soluble iodinated compound should be used. As a general rule, images produced with water-soluble contrast agents are less informative than barium studies, because the water-soluble agents are less dense than barium, do not adhere as well to mucosa, and result in poorer contrast.
In MRI, standard iodinated contrast agents are of no use. Instead, we use magnetically active compounds such as gadolinium or other metals such as iron oxide with unpaired electrons (paramagnetic effects) to enhance imaging certain disease processes. Gadolinium does not produce an MR signal but does cause changes in local magnetic fields by inducing T1 shortening in tissues where it has localized. It is useful for imaging tumors, infections, and acute cerebral vascular accidents. Although the principles of MRI and CT differ, the practical outcomes are similar. They both cause lesion enhancing or in other words a lesion is whiter than the surrounding tissues (Fig. 1.7).
Gadolinium generally has a low risk for reactions and/or nephropathy, but it can cause a severe connective tissue disorder, nephrogenic sclerosing fibrosis (NSF). NSF virtually only occurs in patients who are on dialysis or have a creatinine clearance less than 30 mg/dL. This disease is a very serious complication and is similar to scleroderma. The takeaway lesson on gadolinium is to consult with your radiologist on any patient with known renal failure or a history of NSF before requesting a contrast-enhanced MRI examination.
FIGURE 1.7. Sagittal, coronal, and axial anatomic planes.
COMPUTED TOMOGRAPHY
CT involves sectional anatomy imaging or anatomy in the sagittal, coronal, and axial (cross-sectional, transverse) planes. These terms, which can be confusing, are clearly illustrated in Figure 1.7. Sectional anatomy has always been important to physicians and other healthcare workers, but the newer imaging modalities of CT, MRI, and ultrasonography (US) demand an in-depth understanding of anatomy displayed in this manner.
CT, sometimes referred to as computerized axial tomography (CAT) scan technology, was developed in the 1970s. The rock group, The Beatles gave a big boost to CT development when it invested a significant amount of money in a business called Electric Musical Instruments Limited (EMI). It was EMI engineers who subsequently developed CT technology. Initially, EMI scanners were used exclusively for brain imaging, but this technology was rapidly extended to the abdomen, thorax, spine, and extremities.
CT imaging is best understood if the anatomic site to be examined is thought of as a loaf of sliced bread; an image of each slice of bread is created without imaging the other slices (Fig. 1.8). This is in contradistinction to a radiograph, which captures the whole loaf of bread as in a photograph.
The external appearance of a typical CT unit or machine is illustrated in Figure 1.9. CT images are produced by a combination of x-rays, computers, and detectors. A computer-controlled couch transfers the patient in short increments through the opening in the scanner housing. In the original, now near-extinct standard CT unit, the x-ray tube located in the housing (gantry) rotates around the patient, and each anatomic slice to be imaged is exposed to a pencil-thin x-ray beam (Fig. 1.10). Each image or slice requires only a few seconds; therefore, breath-holding is usually not an issue. The thickness of these axial images or slices can be varied from 1 to 10 mm depending on the indications for the study. For example, in the abdomen and lungs we commonly use a 10-mm slice thickness because the structures are large. A slice thickness of only a few millimeters is used to image small structures like those found in the middle and inner ear. An average CT study takes approximately 10 to 20 minutes depending on the circumstances.
FIGURE 1.8. Illustration of how CT technology creates an image of a single slice of bread from a loaf of sliced bread without imaging the other slices.
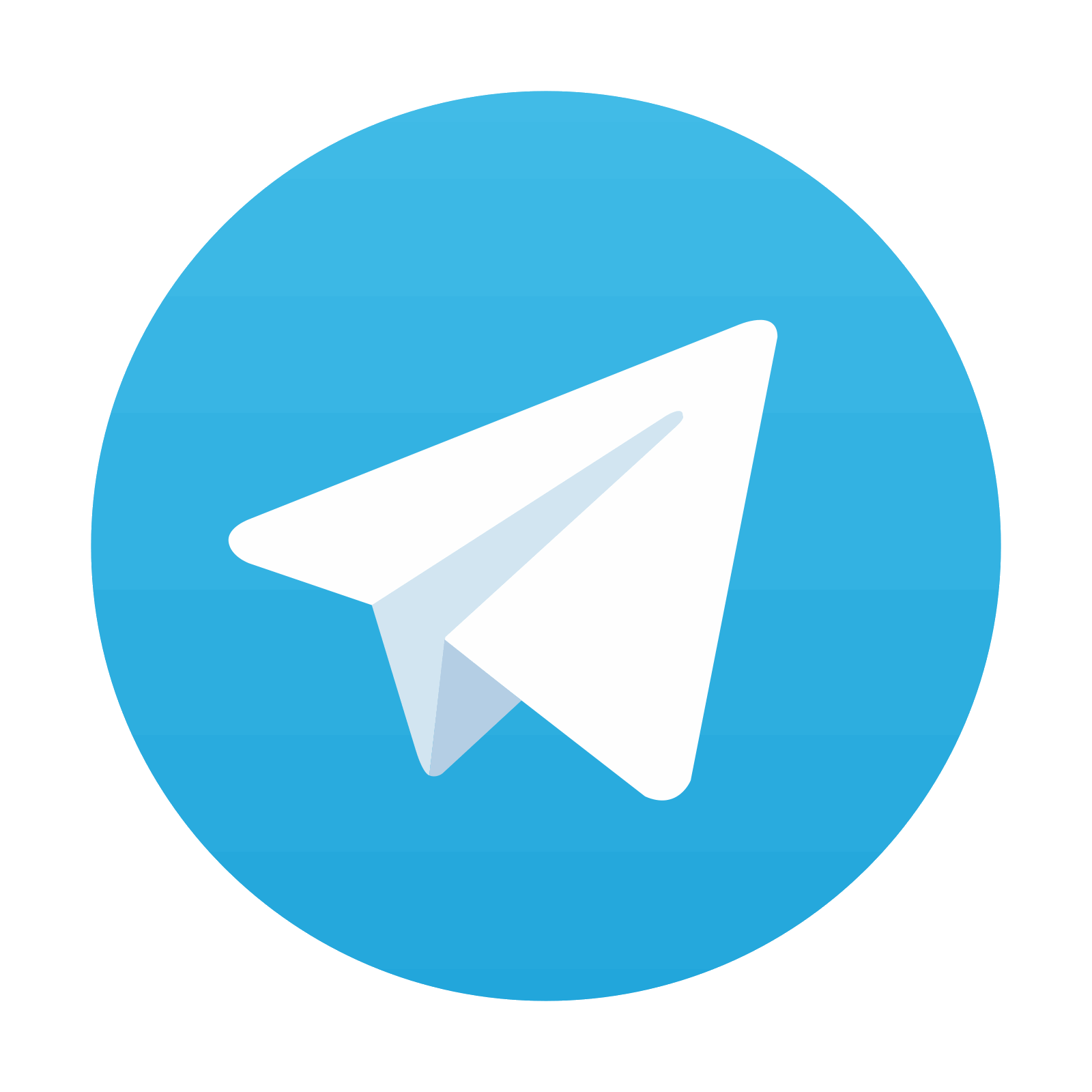
Stay updated, free articles. Join our Telegram channel
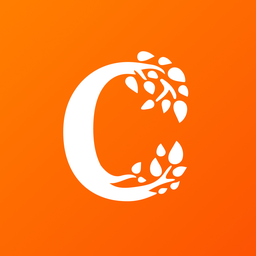
Full access? Get Clinical Tree
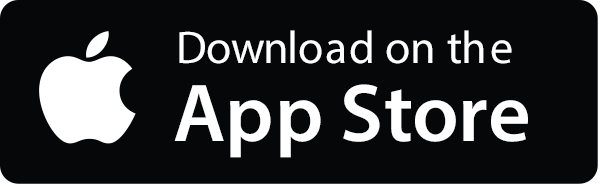
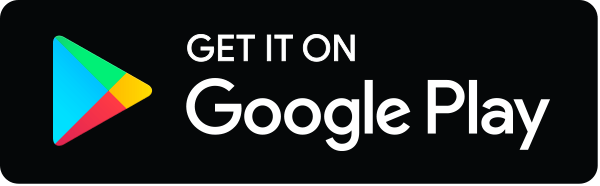