12 Molecular Imaging: PET and SPECT
12.1 Introduction
In the last decade there has been great interest in the clinical application of molecular imaging of tumors following the success of positron-emission tomography (PET) using 18F-fluorodeoxyglucose (FDG) in clinical oncology. Although FDG is extensively and successfully used in different cancers, its sensitivity and specificity are not optimal for all cancer types. FDG evaluates only glucose metabolism of tissue. Numberous PET tracers have been developed to investigate other aspects of tumor physiology in addition to glucose metabolism and are very helpful in detecting tumors, identifying tumor grades and prognosis, guiding and monitoring treatment, and differentiating tumor recurrence from treatment related changes. Many of these radiotracers are at various stages of translation to clinical practice in the United States and have already been in clinical practice in other countries.
In addition to development of newer radiotracers, there has also been tremendous improvement of the molecular (single photon emission computed tomography [SPECT] and PET) imaging instrumentation and reconstruction algorithms allowing imaging with spatial resolution of as little as 3 mm in clinical PET systems.1 There has been remarkable improvement in temporal resolution as well, allowing dynamic PET acquisition. A PET magnetic resonance imaging (MRI) system has been introduced for clinical use and has tremendous potential in oncological imaging.2
12.2 Molecular Imaging: Concepts
Molecular imaging has been defined as “visualization, characterization, and measurement of biological processes at the molecular and cellular levels in human and other living systems.”3 Imaging techniques available for this purpose include nuclear medicine techniques, advanced functional MRI techniques, optical imaging, ultrasonography, and other nanoimaging technologies. The goals of molecular imaging include improved understanding of tumor biology (cancer development, progression, angiogenesis, metastasis, etc.), visualization and noninvasive quantification of different cellular receptors that are typically activated/suppressed in the process of tumorigenesis, studying pharmacokinetics and pharmacodynamics of novel anticancer drugs, and noninvasive quantification of response to predict outcomes in response to such novel therapies.4 Molecular imaging thus differs greatly from the conventional anatomical imaging that is still predominantly used to characterize structural abnormalities, which often correspond to the end result of molecular processes at the cellular and subcellular levels.
12.3 Molecular Imaging: Need in Brain Tumors
MRI is the workhorse in neuro-oncology. In the last 2 decades, there has been significant improvement of MRI instrumentation and development and validation of several MRI-based estimations of tumor physiology, such as diffusion imaging, perfusion imaging, and MR spectroscopy. Even with the availability of these advanced MRI techniques, there are several unmet clinical needs, particularly in detection of actual tumor margin and treatment monitoring. The limitations of MRI become prominent following the clinical availability of increasingly popular antiangiogenic drugs for brain tumor therapy. Antitumor drugs are being developed that target different molecular/genetic events of gliomas, which necessitates accurate and precise molecular imaging technology.
12.4 Molecular Imaging: Instrumentation
12.4.1 SPECT
Brain SPECT has been pursued since the early 1960s. Brain SPECT allows three-dimensional (3D) acquisition of functional information with high sensitivity and specificity. When compared with PET, SPECT provides lower sensitivity, poorer resolution, and lower potential for quantification, and it is uncommonly used in the practice of neuro-oncology. SPECT, however, remains more widely available and is less expensive than PET.
Unlike computed tomography (CT), the source of radioactivity is the patient. The patient is injected with radioactive material that selectively binds to the area of interest and acts as a source of radiation, which is captured using detectors. SPECT systems use collimators to allow only photons approaching at certain angles to reach the detectors from the body part being imaged. To maximize resolution, collimators must be kept as close as possible to the patient. In brain imaging, this means that the collimator must clear the bed and the shoulders of the patient. Head holders are used for brain SPECT and provide comfort while limiting patient motion.
Information from the captured photons is used to reconstruct images using different computational algorithms. Filtered back projection (FBP) with various filters or iterative reconstruction is routinely used to reconstruct images in SPECT. Iterative reconstruction has advantages over FBP in that it allows better control of noise, scatter, attenuation, and other factors affecting image quality. SPECT images can be fused to a separately acquired CT scan or can be obtained in dedicated SPECT/CT scanners.5
12.4.2 PET CT
PET imaging relies on the nature of the positron and positron decay. The positron is the antimatter counterpart to the electron and therefore has the same mass as the electron but the opposite charge.6 Positron decay can be explained with the equation:
where, (P+) is a proton, N is a neutron, (β+) is a positron, ν is a neutrino, and E is excess energy released. Positrons are emitted from the atomic nucleus, and as they pass through matter they travel a short distance and interact with an electron via annihilation. From this interaction, two photons are emitted, each with an energy of 511 keV, in opposite directions of each other. Simultaneous emission of annihilation photons traveling 180 degrees from each other forms the basis of PET imaging, through coincidence detection and coincidence imaging.6 The detector in a conventional PET scanners consists of a ring of scintillation crystals in a 360 degree array. The basic principle of coincidence imaging is that two photons detected in close temporal proximity by two opposed detectors in the ring are likely to be from a single annihilation event. When simultaneous detection of two photons is registered at opposite directions of each other within the ring, it can be assumed that the annihilation event took place somewhere on a line (line of response [LOR]) between the two detectors.6 When LORs are combined, they represent all of the coincidence events for a given acquisition, which can be depicted as a sinogram. An extremely fast and accurate electronic circuit is required to identify whether two detected photons are the result of a single coincidence event and to analyze the sonogram for quick image reconstruction.
Events detected by PET scanners can be true, scattered, or random events, all of which may be recorded as coincidence events, provided that both annihilation photons are actually detected by opposite detectors within the coincidence window. However, only true coincidence events provide the desired information needed for accurate images of the distribution of the positron emitting radiopharmaceutical for clinical imaging. Because coincidence imaging requires registration of two photons produced during a single annihilation reaction, attenuation of photons at the time of traveling toward detectors markedly impact the final raw data set. Attenuation correction is, therefore, an extremely important step in PET imaging.6,7
Attenuation correction can be performed by either calculated or measured methods. The calculated method is no longer used in current systems. On current hybrid PET/CT scanners, a low-dose CT scan is obtained prior to positron-emission imaging, which yields information used to create an attenuation map for attenuation correction of the PET raw data set. Positioning for the low-dose CT scan needs to be the same as for the positron-emission imaging. It is important to account for factors that may alter the attenuation map because these can introduce alterations in the data that can translate into artifacts in the final corrected images. In addition to attenuation correction, CT is also used for accurate anatomical localization of increased tracer accumulation in tissues.7
Images on a PET scanner can be acquired by using either two-dimensional (2D) or 3D techniques. Two-dimensional imaging uses an axial collimator made of thin septa of lead or tungsten placed between detectors, such that coincidence events are accepted by only adjacent detectors in a ring. This allows for a defined plane or slice and eliminates out-of-plane scatter. Two-dimensional imaging is usually performed when imaging small structures or limited fields of view, such as in brain imaging. Two-dimensional acquisitions provide better image quality, although sensitivity is reduced secondary to collimation.7
Three-dimensional acquisition is volume based and allows for imaging of larger structures or fields of view. When compared with 2D techniques, 3D has higher sensitivity because it allows registration of more coincidence photons, but image quality is reduced as a result of a larger amount of scatter. Three-dimensional imaging is commonly used when imaging small fields of view and areas of low scatter, such as imaging of the brain. Final image processing will require converting the emission data into an image format, either by FBP or using iterative reconstruction.7
PET allows semiquantification of the activity accumulated in tissue at a particular location. This measure is called standardized uptake value (SUV), and it is defined as the image-based concentration of the positron emitter in tissue, divided by the injected dose and multiplied by a calibration factor. Usually the maximal activity is determined from the pixel with highest concentration of the radiopharmaceutical. Semiquantitative determination of tracer accumulation in lesions allows a more objective tool to assess tumor physiology as well as a tool to follow lesions over time and to monitor therapy effects.
12.4.3 PET-MRI
A PET-MRI system has been introduced for clinical use. There are two main imaging approaches in PET-MRI, based on either sequential or simultaneous acquisition of the PET and the MRI data. A sequential system is simple sequential acquisition of PET and MRI data that are later fused through coregistration. Performing simultaneous PET and MRI acquisition poses multiple technical challenges but provides advantages over the sequentially acquired studies, including optimal image registration, correction of the PET data for motion during the scan, and MR-guided PET image reconstruction. Simultaneously acquired studies have markedly improved spatial resolution and allow a unique opportunity for studying transient phenomena with both modalities, a unique advantage of this approach.8
12.5 Molecular Imaging: Glioma
12.5.1 Imaging of Tumor Physiology
Evaluation of Tumor Proliferation
Uncontrolled cellular proliferation is the key pathophysiologic abnormality of cancer. The rate of cell division is an important characteristic of malignancies, particularly in glioblastoma multiforme (GBM). Even though the percentage of cellular proliferation has not been included in the diagnostic criteria of higher grade gliomas in the most recent World Health Organization (WHO) definition, the percentage of tumor cells in the active cell cycle (as identified by the MIB-1 score) is an important biomarker of tumor physiology for high-grade gliomas and correlates well with tumor cell density, histopathological grade of the tumor, and, most importantly, survival time.9 The ability to assess the cell proliferation rate of tumors by noninvasive imaging markers can improve diagnosis, grading, and staging of cancers as well as being useful for longitudinal follow-up and prediction of tumor response to anticancer drugs.10The most commonly used PET tracer for evaluation of tumor proliferation is: (3′-18fluoro-3′-deoxy-L-thymidine [FLT]).
DNA replication is the key prerequisite for cell division. Thymidine is one of the four nucleosides required for DNA synthesis, which can be synthesized de novo within the cell by thymidylate synthase or can be obtained through phosphorylation of thymine deoxyriboside (TdR) by thymidine kinase (TK) through the so-called salvage pathway. Expression of DNA synthesis enzymes is a highly regulated process and depends on the cellular cycle. The expression of TK1 increases as much as 10 to 20 times the baseline in G1/S phase transition, remains high in S, G2, and M phases, and then rapidly declines as the cell approaches the G0 to G1 phase.10 TK2, another isoform of TK, is constitutively expressed, and expression of TK2 is not dependent on cell cycle phase.10
FLT is a thymidine nucleoside analogue that was originally developed as an anticancer and antiretroviral agent. Due to the high incidence of side effects, it has never been approved for clinical use. However, experience from clinical trials forms the background of using the compound as a PET tracer. The principle mechanism of FLT PET is uptake of FLT by proliferating tumor cells, mostly in the S phase.11 Inside the cell, FLT is phosphorylated by TK1 to an intermediary metabolite that is resistant to degradation and phosphorylated, as a result of which FLT is trapped inside proliferating cells.10 The rate-limiting step in FLT accumulation is phosphorylation by TK1; therefore, the intracellular level of phosphorylated FLT indirectly reflects TK1 activity that has also been shown to strongly correlate with thymidine incorporation into DNA.12,13 Over time, there is a very slow efflux of FLT due to dephosphorylation, allowing a significant period of relatively stable tracer retention inside cells for imaging.
The injected does of FLT is based on body weight, and the usual dose is 2.7 MBq/kg with a maximum dose of 185 MBq. There are two different approaches to analyze FLT PET imaging. The first is the standard SUV-based method as used in FDG PET. The other method uses dynamic imaging sequence and kinetic modeling of tracer uptake. This approach is unique in the sense that it can quantify FLT uptake and retention over time and estimate the rates of underlying biological processes.
Evaluation of Tumor Metabolism
Living cells require a relentless supply of essential nutrients. Transmembrane transport and biosynthesis generate and maintain an internal pool of these essential molecules for internal metabolism. Transports of nutrient molecules are tightly regulated and largely depend on the demand of the cell at a given time. Therefore, imaging strategies that can noninvasively image the nutrient uptake can provide insight about the real-time metabolism of the cell.
Glucose Metabolism
Malignant tumors have higher rates of glucose usage and glycolysis. Based on this knowledge, FDG has been developed and is being successfully used to evaluate numerous cancer types. FDG differs from glucose only for the replacement of a hydroxyl group with radioactive fluorine. FDG actively enters cells via the same group of carrier-mediated active transport molecules (glucose transporter 1 [GLUT1]) as for glucose. Similar to glucose 18FDG undergoes phosphorylation within the cell by hexokinase to FDG-6-phosphate, which cannot be metabolized rapidly. Essentially FDG-6-phosphate is trapped inside cells. Therefore imaging with FDG allows noninvasive assessment of the rate of glucose uptake/ requirement of a cell.
There is upregulation of expression of GLUT1 as well as hexokinase in tumor cells because tumor cells use a large amount of glucose as the only energy source. As a result, uptake of FDG is increased in tumor cells. Normal neurons in brain also depend heavily on glucose as a source of energy to maintain high energy-consuming synaptic activity. Expectedly there is also high FDG uptake in normal brain, particularly in the neuron-rich gray matter. This explains significantly lower tumor-to-background contrast, even in high grade central nervous system (CNS) tumors and limits the use of FDG in evaluation of brain tumors.
Several processes determine FDG uptake in tumor cells. The most important factor is the intact blood supply for delivery of the tracer into tumor cells. The number of tumor cells and degree of cellular proliferation also increase uptake of FDG. Uptake of FDG in the hypoxic area of a tumor depends on a complex interaction between the tumor microenvironment and the blood supply. In hypoxic areas there is less delivery of FDG; however, uptake of FDG in tumor cell is very high due to hypoxia-inducible factor 1-alpha (HIP1-α) further upregulation of GLUT1 and hexokinase.14 A necrotic area of a tumor does not uptake FDG for obvious reasons. Steroid use may also interfere with FDG uptake in tumor cells.15
There are several limitations of FDG in evaluation of brain tumors. First, the sensitivity of detection of low-grade gliomas using FDG PET is low due to the relatively small differences in the rates of glucose usage in tumor cells and normal brain cells.16 Increased FDG uptake is not specific for tumor cells. Inflammatory cells, particularly macrophages, also intensely uptake FDG, even inside a tumor.17 As a result, high FDG uptake by a lesion is not tumor specific, and FDG PET has demonstrated low sensitivity in differentiation treatment effects from recurrent glioblastoma.18,19 Finally, it is difficult to precisely delineate the tumor margin if the tumor is located close to the basal ganglia or close to the cortex because of high FDG uptake in normal gray matter.20
Amino Acid Metabolism
Due to the inherent limitations of FDG PET, the use of alternative tracers has been proposed for evaluation of metabolism of brain tumors. In this regard, imaging with radiolabeled amino acid is a brilliant concept. Amino acid imaging allows excellent contrast between tumor and background normal brain because there is very limited uptake of amino acids by normal brain tissue. This allows easy tumor detection and better delineation of tumor margin in comparison to FDG. Amino acids are transported inside the cell by specific transporter systems and are essential cellular nutrients, particularly in rapidly dividing cells.21 Amino acid uptake depends on the flux of amino acids to the tissue, expression of amino acid transporters, and rate of amino acid metabolism. In animal models increased amino acid uptake has been linked to upregulation of amino acid transport in the supporting vasculature of the tumor regardless of the phase of the cell cycle and even in the absence of increased vascular permeability.22,23 These results indicate that tumor uptake of amino acids does not depend on breakdown of the blood–brain barrier.24
L-(methyl-11c) Methionine (Met)
Met has been most extensively studied in evaluation of brain tumors, and in many European countries this is the most popular amino acid PET tracer routinely used for brain tumor evaluation. High uptake of Met is related to increased amino acid transport mediated by L-type amino acid transporter and has been shown to correlate with in vitro cell proliferation, Ki 67 nuclear antigen expression, and microvessel density in primary brain tumors, suggesting that this could be a biomarker for tumor proliferation as well as angiogenesis.20 Unlike FDG, Met is accumulated mostly in viable cancer cells with minimum uptake in macrophages and other nonspecific cellular components of the tumor.17 Significant uptake is seen in low-grade tumor as well, although the uptake in low-grade tumors is lower in comparison to high-grade tumors. The major limitation of this tracer is very short half-life (only 20 minutes).
2-18F-fluoroethyl)-L-Tyrosine (FET)
FLT is a fluoro-alkylated analogue of another essential amino acid, L-tyrosine. During the last 2 decades more and more studies have been performed with FET PET, mainly because of the longer half-life of 18F (t1/2 = 110 min) in comparison to the shorter half-life (t1/2 = 20min) of 11C, allowing imaging even without an on-site cyclotron. It does not accumulate in normal brain tissue, but uptake is higher in tumor tissue. Multiple studies28,29,30 have demonstrated that FET PET is better than FDG PET in showing markedly elevated uptake in both high- and low-grade gliomas. It has been shown that diagnostic performance of FET PET as a tumor marker in gliomas and metastatic brain tumors is similar to that of Met PET, with a sensitivity of 91% and s specificity of 100%.28
3,4-dihydroxy-6-[18F]-fluoro-L-phenylalanine (FDOPA)
Although the mother compound of FDOPA is not an amino acid, it is a product of an essential amino acid, L-tyrosine, and is considered as amino acid analogue. Tumor-to-background contrast is very good because of elevated amino acid transport in malignant brain tumors.
Oxidative Metabolism
Acetate is one of the most common building blocks for biosynthesis in the living organisms. In tumor cells, most of the acetate is converted into fatty acid synthetase and is predominantly incorporated into intracellular phosphatidylcholine membranes. Due to this feature, 11C acetate (ACE) can serve as a PET tracer.
Evaluation of Angiogenesis and Invasion
Tumor neovascularization, the formation of new blood vessels from preexisting vasculature, plays a vital role in tumor growth and is recognized as a key event in the natural progression of gliomas.29 Tumor neovascularization is an extremely complex tumor physiology and is the end result of the interaction of hundreds of pro- and antiangiogenic molecules. At least five different mechanisms have been described in GBM, of which angiogenesis is the predominant mechanism.30
Activated endothelial cells expresses αvβ3, a dimeric transmembrane integrin that interacts with extracellular matrix protein and regulates migration of endothelial cells through the extracellular matrix during neoangiogenesis.31 Additionally, αvβ3 and different other integrins are also expressed by glioma cells that function at almost every step of tumorigenesis, including development and progression of gliomas,32 tumor cell migration, and invasion.33,34,35 αvβ3 binds to arginine-glycine-aspartic acid (RGD) containing motif of the interstitial matrix proteins such as vitronectin, fibronectin, and thrombospondin.36,37 Based on this observation, both linear and cyclic RGD compounds have been introduced for imaging and have been shown to have high binding affinity and selectivity to the αvβ3 integrin. Numerous chemical modifications have been suggested for better pharmacokinetic profiles of the RGD-containing molecules. Similarly, several different radionuclides have been also been used to tag these molecules in order to optimize imaging properties. The most commonly used radionuclide is 18-Fluoride. It is not known yet proved whether RGD imaging actually images αvβ3 expression by the endothelial cells or by the glioma cells. Using a human glioma cell line in mouse models Battle et al have demonstrated that 18Fluciclatide (a PET imaging agent with an RGD sequence) can detect changes in tumor uptake of this PET tracer after acute antiangiogenic therapy.38
Vascular Endothelial Growth Factor
Vascular endothelial growth factor (VEGF) is one of the key regulators in glioma angiogenesis. Among the VEGF gene family, VEGF-A is a homodimeric glycoprotein that has seven different isoforms with varying numbers of amino acid length.39 All isoforms of VEGF-A bind to both the VEGF receptors family: VEGFR1 and VEGFR2. Binding to VEGFR2 plays an orchestrating role in angiogenesis.39 PET imaging probes that specifically bind to the VEGF or VEGFR2 can provide a tumorwide angiogenesis profile that can serve as a more precise assessment of tumor angiogenesis and can be used to assess the effects of antiangiogenic therapy. It has been shown that there is rapid, prominent, and specific uptake of a new 64Cu labeled DOTA-VEGF121 (VEGF-A isoform with 121 amino acids) PET probe in the U87MG cell line with high expression of VEGFR2 in comparison to the same U87MG cell line with low VEGFR2 expression.40,41 It has also been shown that this particular PET tracer is able to demonstrate the dynamic expression profile of VEGFR2 expression at different stages of tumor.40
Evaluation of Hypoxia
FMISO
In GBM, tumor cell growth outstrips the vascular development. As a result, oxygen delivery is decreased beyond its diffusion distance, and there is hypoxia in the tumor microenvironment.42 Hypoxia is a predominant feature of the microenvironment of higher-grade gliomas, particularly in GBM. It has been well established that hypoxia is associated with tumor growth, progression, angiogenesis, invasion, increased cancer stem cell population, genomic instability, resistance of conventional therapies, recurrence, and decreased patient survival.43,44 Hypoxia-inducible factors (HIFs) is the master regulator of the aforementioned tumor biology acting as a transcription factor and activating hundreds of downstream genes.45 Active research has been ongoing for accurate imaging of hypoxia using immunohistochemistry, MRI, PET, and SPECT. Several PET and SPECT tracers have been investigated to quantify tumor hypoxia.
Nitroimidazole Agents
3-18Fluoro-1-(20-nitro-10-imidazolyl)-2-propanol (FMISO)
FMISO is the most extensively investigated and validated PET radiotracer for hypoxia imaging to date.46 FMISO is a nitroimidazole compound that undergoes reduction into a reactive intermediary metabolite within a living cell. Uptake of 18FMISO is inversely proportional to O2 level, its delivery to the tumor core is not dependent on tumor perfusion, and the molecule diffuses freely into all cells. In normoxic cells (where electron transport is occurring), the NO2 subunit of FMISO takes an electron to form a radical anion reduction product, then rapidly transfers the electron to O2 and returns to its original structure. However, in hypoxic cells the molecule undergoes further reduction, forms covalent bonds to intracellular macromolecules, and is thereby trapped inside the cell.42,47 It is not retained in necrotic tissue because there is no electron transport.47 Several studies have proved that uptake of FMISO directly correlates with tissue oxygenation level.48,49,50 Binding of FMISO to tissue is dependent on the level of hypoxia, and oxygen levels of 3 to 10 mm Hg lead to FMSIO binding.51,52 More hypoxic tissue binds FMISO more avidly. Because there is no uptake of FMISO in normoxic brain cells, FMISO PET allows excellent contrast between the tumor and normal brain.42
There is a longer waiting period (90–140 min) between injection and the start of the scan for FMISO in comparison to FDG because of slower clearance kinetics, low uptake in hypoxic cells, and the absence of any active transport system for FMISO.53 The usual injection dose is 3.7 MBq/kg with a maximum dose of 260 MBq.42 Typically, image analysis is performed after coregistration of the PET data with contrast-enhanced 3D MRI to delineate tumor margins and to identify the regions of interest. Typically, the degree of radiotracer uptake of tumor is compared with the venous blood sample after decay time correction. FMISO image data are divided with the blood radiotracer level to generate the pixel-by-pixel tissue/blood (T/B) level map.54 T/B > 1.2 is considered significant hypoxia and is usually used to generate a hypoxic volume map.
Imaging in very early stage, FMISO distribution reflects tumor blood flow, whereas later on this mostly accumulates in hypoxic cells with a very high tumor-to-background ratio. The metabolites of FMISO rapidly clear from plasma, allowing no need of normalization for delivery. FMISO has no protein binding, and relatively early imaging is possible. Additionally FMISO has been validated in numerous animal models and human disease conditions, and its signal is independent of other tumor-related factors, such as regional glucose consumption, glutathione level, and local pH.
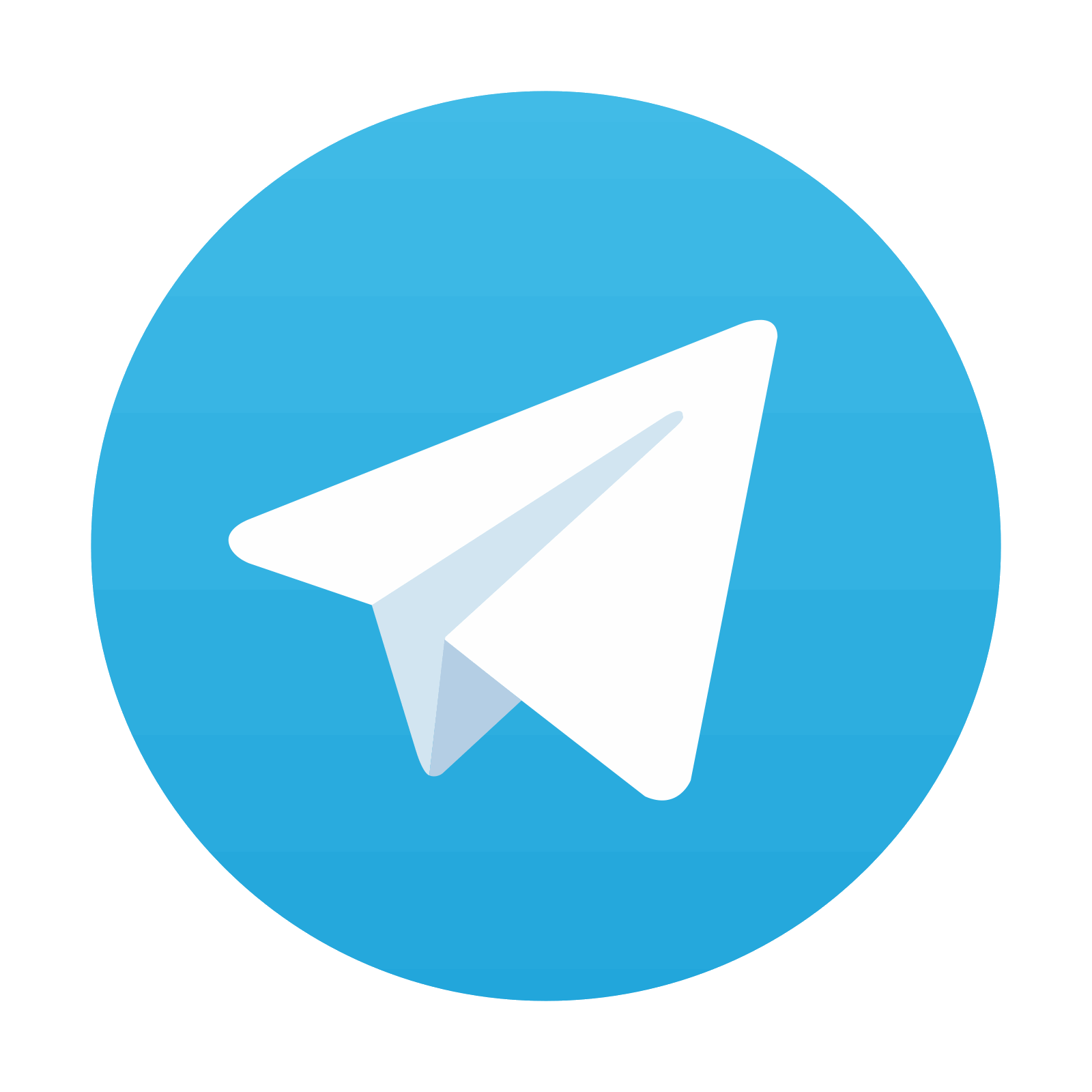
Stay updated, free articles. Join our Telegram channel
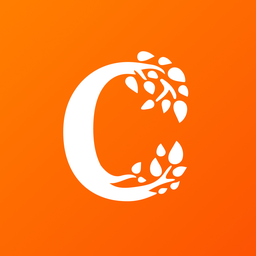
Full access? Get Clinical Tree
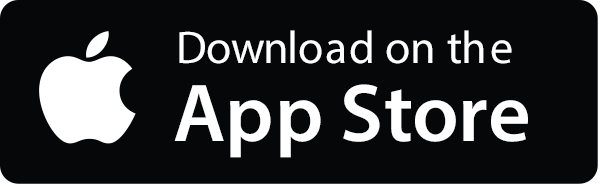
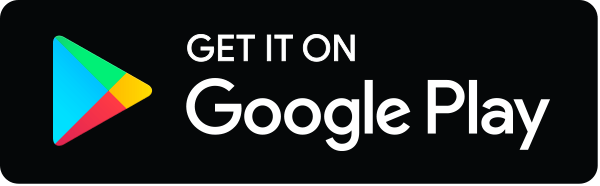
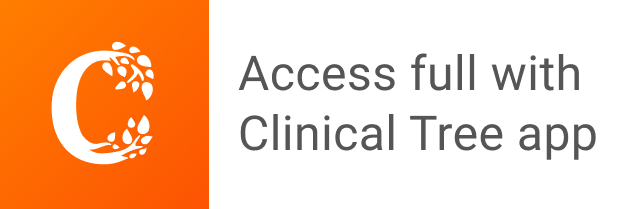