- Basic physics of ionizing radiation
- Types of ionizing radiation and their characteristics
- Units for quantifying radiation, including traditional and Système International (SI) units
- The purpose and use of each radiation unit
Introduction
Radiation is energy transmitted through a medium as either electromagnetic (EM) waves or subatomic particles. Larger particles (e.g., molecules, pollen, raindrops, bowling balls) may be capable of transmitting energy, but they do not qualify as radiation. The medium through which radiation is transmitted may be vacuum, air, tissue, or some other material. In this course, we are especially concerned with what happens when radiation is transmitted through the cells and tissues of living organisms.
Ionizing radiation is radiation with sufficient energy that it can knock some of the electrons out of the atoms or molecules in a material that it passes through. The process of changing the number of electrons in an atom (adding or removing) is referred to as ionization; an atom with unequal numbers of protons and electrons is an ion.
When ionization occurs in living tissue, the electron may quickly recombine with its atom, and little damage may occur; but in some cases, significant damage at the cell, organ, or organism level may occur. A fundamental process caused by ionizing radiation is the splitting of atoms or molecules into positively and negatively charged fragments. These ions may then participate in chemical reactions, resulting in chemical changes that can lead to disruptions of cellular function in living tissue. Ionizing radiation comes from a variety of sources, including cosmic rays, natural radioactivity in the soil and in building materials, industrial sources (e.g., airport scanners), and medical devices (e.g., diagnostic X-ray or radiation therapy machines).
Many people fear radiation for several reasons: it cannot be seen, smelled, heard, or felt; it can cause biological damage; journalists like to write about the potential dangers of radiation (with a frequency and fervor that is often out of proportion to the harm it actually causes). In many ways, radiation is similar to electricity, but we have become accustomed to living with electricity in our homes and offices. Although electricity and automobiles (not to mention tobacco and ethanol) cause many more injuries and deaths each year than radiation, we tend to fear the unknown. Most people have no experience with radiation, beyond UV exposure from the sun and the occasional medical X-ray. Therefore, the average person is more frightened of radiation than electricity, because the average person knows something about how electricity is used and controlled (e.g., it can be shut off with a switch, “live” wires can be insulated with a plastic coating). Some sources of radiation (nuclear reactors, X-ray machines) can also be shut off, but the radiation we most fear comes from radioactive materials (radioactive waste generated in nuclear power plants, nuclear materials in weapons). Although radioactive materials cannot be turned off, simple measures, such as the use of shielding materials and maintaining a safe distance, are very effective at avoiding harmful radiation exposure.
One good property of radiation is that it is very easy to detect. Ionizing radiation passing through air creates an electrical charge that is easy to measure. Many of our medical colleagues wish there were a meter or detector for common diseases, such as cancer or Alzheimer’s, which was as sensitive and readily available as radiation detectors.
The details of how radiation interacts with matter in general and biological matter in particular, will be the topic of later chapters. In this chapter, we will introduce the types of ionizing radiation and discuss their characteristics. We will also introduce the units of measurement used to quantify radiation.
Types of Ionizing Radiation
There are two basic types of ionizing radiation: EM radiation and particulate radiation. Briefly, EM radiation involves the transmission of energy, but not mass. In contrast, particulate radiation consists of subatomic particles having both mass and energy. Importantly, both EM and particulate radiation with sufficient energy are capable of tearing electrons out of atoms or molecular bonds in matter.
EM Radiation
EM energy has both wave- and particle-like characteristics. The wave description is more useful for describing EM energy propagating through space or a medium. Figure 3.1 illustrates the wave-like properties of radiation. Like any traveling wave, a traveling EM wave will have a propagation speed (v, in units of m/s), a wavelength (λ, in units of m), and a frequency (f, in units of s−1). In a given medium, the speed is constant, so a wave with higher wavelength will have a lower frequency, where v = λf.
Figure 3.1 For an electromagnetic wave, the wavelength is defined as the distance between adjacent peaks.
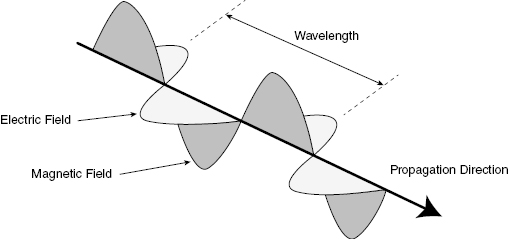
On the other hand, when EM energy interacts with matter, it is absorbed or emitted in discrete amounts (i.e., quanta), as if it were a particle. The quantum of EM energy is called a photon. Calling a photon a “particle” is somewhat misleading, since photons have no mass. Because EM radiation possesses both wave- and particle-like properties simultaneously, the energy E of an absorbed or emitted photon is related to the wavelength in a simple way:
(3.1)
where h is Planck’s constant (h = 6.626 × 10−34 J-s). The Système International (SI) unit of energy is the joule (J), but radiation energies are more conveniently expressed in electron volts (eV), where 1.602 × 10−19 J = 1 eV. An electron volt is the amount of kinetic energy gained or lost when a single electron moves through a potential difference of 1 V. As an example, an electron moving from the positive to the negative terminal of a 9-V battery will gain 9 eV of energy. An electron volt is an extremely small unit of energy, compared with energy scales used to describe ordinary objects and processes. A donut contains about 60 mJ or 3.7 × 1017 eV of stored energy. A 60-W lightbulb is expending more than 3 × 1020 eV (300 trillion MeV) per second. Nevertheless, the energy of a few dozen electron volts, deposited in an atom in tissue, is a potentially significant event in radiation biology. The energy of EM radiation extends from lower-energy radio waves to high-energy gamma rays. Figure 3.2 presents portions of the EM spectrum from the low-energy, long wavelength end to the high-energy, short wavelength end.
Figure 3.2 The electromagnetic (EM) spectrum, showing the corresponding wavelength, frequency, and energy scales. Note that shorter wavelengths correspond to higher frequencies and energies. Visible light occupies only a small portion of the EM spectrum and is near the upper end of the nonionizing energy range.
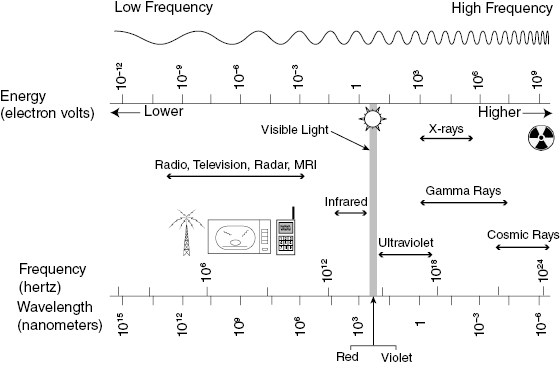
Infrared and visible light are forms of EM radiation, encountered every day, which cause some biological effects. We feel heat (mainly due to photons in the infrared energy range), and we see using visible light, both of which are biological effects. However, infrared and visible photons are not energetic enough to cause ionization (i.e., eject orbital electrons from atoms). Ionizing EM radiation includes photons with energies of about 10 eV or higher (including the far UV, X-rays, and gamma rays). Ionizing radiation can cause chemical changes that may result in significant biological effects, including cell death or cancer. Figure 3.3 illustrates the ionization of an atom by an incident X-ray.
Figure 3.3 Absorption of a photon of EM radiation by an atom results in the sudden transition of an electron to a higher energy level. Emission of a photon involves the transition of an electron to a lower energy level.
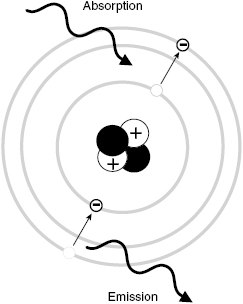
Ionization is a quantum effect—understanding this concept is very important to understanding the biological effects of EM radiation. If an electron requires a 12-eV energy gain in order to be ejected from an atom in some material, then the atom must absorb a single photon of energy 12 eV or greater. To produce ionization, the photon energy must be greater than the energy required to remove an electron from the material. It must be greater than the electron’s binding energy. If there are many 4-eV photons striking the material, one might think that three of them together could eject an electron. But ejecting an electron is not like lifting a Volkswagen—even though one person cannot lift a Volkswagen, several people working together can. Ionization is more like trying to throw a rock across a wide river. If one person cannot throw a rock far enough, and none of his friends can throw a rock far enough, then the whole group can throw rocks at a furious rate, and not a single rock will make it across the river. In the same way, multiple nonionizing photons do not add together to eject an electron from an atom or split a molecule into fragments. While a very high intensity of nonionizing radiation (i.e., many low-energy photons) can cause significant heating, leading to burns or even death, a certain level of nonionizing radiation exposure (i.e., heat) is absolutely necessary for the survival of living organisms. Ionizing radiation is different: it results in fundamentally different mechanisms of biological harm, it appears one can live without it, and it is not clear whether any amount of ionizing radiation is actually good for you.
Note that radiation emitted from cell phones is nonionizing. The energy of photons emitted from a cell phone is lower than the energy of many of the photons coming from a toaster (which emits significant energy in the infrared and even the lower end of the visible spectrum). In the consumer realm, it makes sense to fear tanning beds, which do emit ionizing radiation that will increase one’s risk of melanoma, the most serious type of skin cancer. However, there is no conclusive experimental evidence at this point (2011) that cell phones (or any other devices that emit low-energy photons—such as hair dryers or FM radios) cause cancer. Nonetheless, worrying about cell phone radiation has been a popular topic in the press in the first decade of the 21st century. Similarly, there is no conclusive evidence that living near power lines or power substations causes cancer (a popular topic in the latter decades of the 20th century), for all the same reasons mentioned earlier.
X-rays and gamma rays are the forms of ionizing EM radiation that are the most important to consider for a course in radiobiology applied to medicine. X-rays and gamma rays lie at the high-energy part of the EM spectrum as shown in Fig. 3.3. Note that gamma rays and high-energy X-rays are physically identical. The reason for the different names arises from differences in their origin (inside vs. outside the nucleus). X-rays are produced in the orbital electronic shells of an atom or by interactions between charged particles and the nucleus, whereas gamma rays are emitted from an unstable nucleus after rearrangement of the particles inside the nucleus, as described in more detail later.
As noted earlier, gamma rays and X-rays are photons, which possess zero electrical charge and zero mass, and therefore have a relatively low probability of interacting in matter. As a result, photons can penetrate deeply into matter, because the interactions required to dissipate their energy are infrequent. Photons do carry energy and can ionize atoms or molecules via direct interactions with orbital electrons. They do not have a well-defined maximum penetration depth in matter, but we can define an average penetration depth for a given material. To effectively shield gamma rays and X-rays requires electron-dense materials, for example, steel or lead.
Atoms and Isotopes
The atom is the smallest unit of matter possessing a unique chemical identity. Atoms consist of subatomic particles: positively charged protons and uncharged neutrons occupy a dense nucleus, which is surrounded by a “cloud” of negatively charged electrons.
Atomic nuclei are denoted by the atomic number Z, which is the number of protons in the nucleus, and the atomic mass number A, which is the total number of nucleons (protons and neutrons) in the nucleus. To label nuclei, Z is written to the lower left of the chemical symbol, and A is written to the upper left of the chemical symbol. The nucleus of an element with chemical symbol X would be written . As an example, the carbon-12 nucleus, which has six protons and six neutrons, is written as
The chemical characteristics of an atom are defined by its electron configuration, which is the basis for the assignment of elements to particular columns in the periodic table, shown in Fig. 3.4. Recall that elements in the same column of the periodic table have similar chemical properties. In the neutral atom, the number of electrons equals the number of protons. Therefore, all atoms with the same number of protons in their nuclei have the same chemical characteristics. By definition, atoms with the same number of protons are the same element and are denoted by the same chemical name and chemical symbol (e.g., six protons = carbon = C). Thus, the chemical symbol and the atomic number (Z) are actually redundant, and Z is often omitted when specifying a nucleus (e.g., carbon-12 or 12C). The simplest atom is hydrogen (1H) with one proton in the nucleus and one electron. The most abundant isotope of helium (4He) has two protons and two neutrons in the nucleus.
Figure 3.4 The periodic table of the elements organizes the elements according to their electronic structure. The number in the upper right corner is the number of protons in the nucleus. From Halliday et al. (1994), Appendix E.
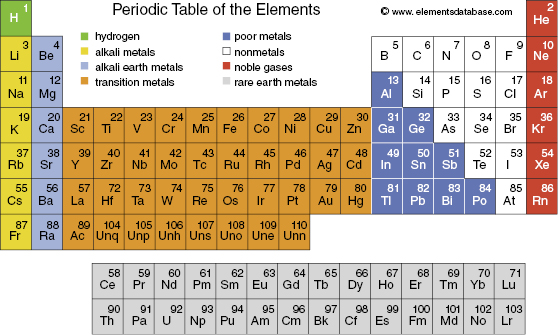
Isotopes have the same number of protons but may have different numbers of neutrons, and therefore different mass numbers. For example, the different isotopes of carbon are written as
means this isotope of carbon has 13 nucleons, which includes six protons, in the nucleus. By subtraction, the number of neutrons (N) in the
nucleus is seven (i.e., 13 − 6 = 7).
The two important forces in the nucleus are ordinary electrostatic repulsion (i.e., the Coulomb force between the positively charged protons) and the strong nuclear force, which is attractive and holds the nucleus together. (Note that there are only four fundamental forces; the other two are the weak nuclear force and gravity.) In order for a nucleus to remain in existence indefinitely, there must be a balance between the repulsive and attractive forces. Such a nucleus is referred to as “stable.” Unstable nuclei will give up energy until they achieve a more energetically stable configuration; energy given up by an unstable nucleus is emitted in the form of particles with high kinetic energies (e.g., a fast-moving electron). Generally, the energies are high enough to cause ionization, which is why we are concerned with nuclear stability in this course.
Energetically stable nuclei tend to have roughly the same number of protons and neutrons. If a nucleus is unstable (too many neutrons or too many protons), it transforms into a new, more stable nucleus by a radioactive decay process. There are many different decay processes, but the ones most relevant to radiobiology involve the emission of particles (alphas, betas, positrons, or neutrons), or the emission of high-energy photons (gamma rays).
Given one unstable nucleus, it is impossible to predict when it will decay. However, given a huge group of identical unstable nuclei, then, statistically, one can predict with high precision how long it will take for a given fraction of the group to decay. Every radioactive isotope decays with a half-life that is constant and unique to that isotope. The half-life is simply the time required for half of the nuclei (no matter how many you have) to decay into another type of nucleus. (So, if the half-life is 1 hour, one can reliably say that half of the nuclei will decay in 1 hour, but one cannot say which particular individual nuclei will be the ones to decay.) The time dependence of the activity of an isotope undergoing radioactive decay is shown in Fig. 3.5. The half-life cannot be altered by any ordinary physical means, for example, by freezing or compressing the substance. As stated earlier, the radiation emitted from radioactive materials cannot be “turned off.” Note that the half-life does not depend on the number of nuclei present.
Figure 3.5 The activity of a radioisotope decays exponentially as a function of time with a rate constant λ according to the equation A/A0
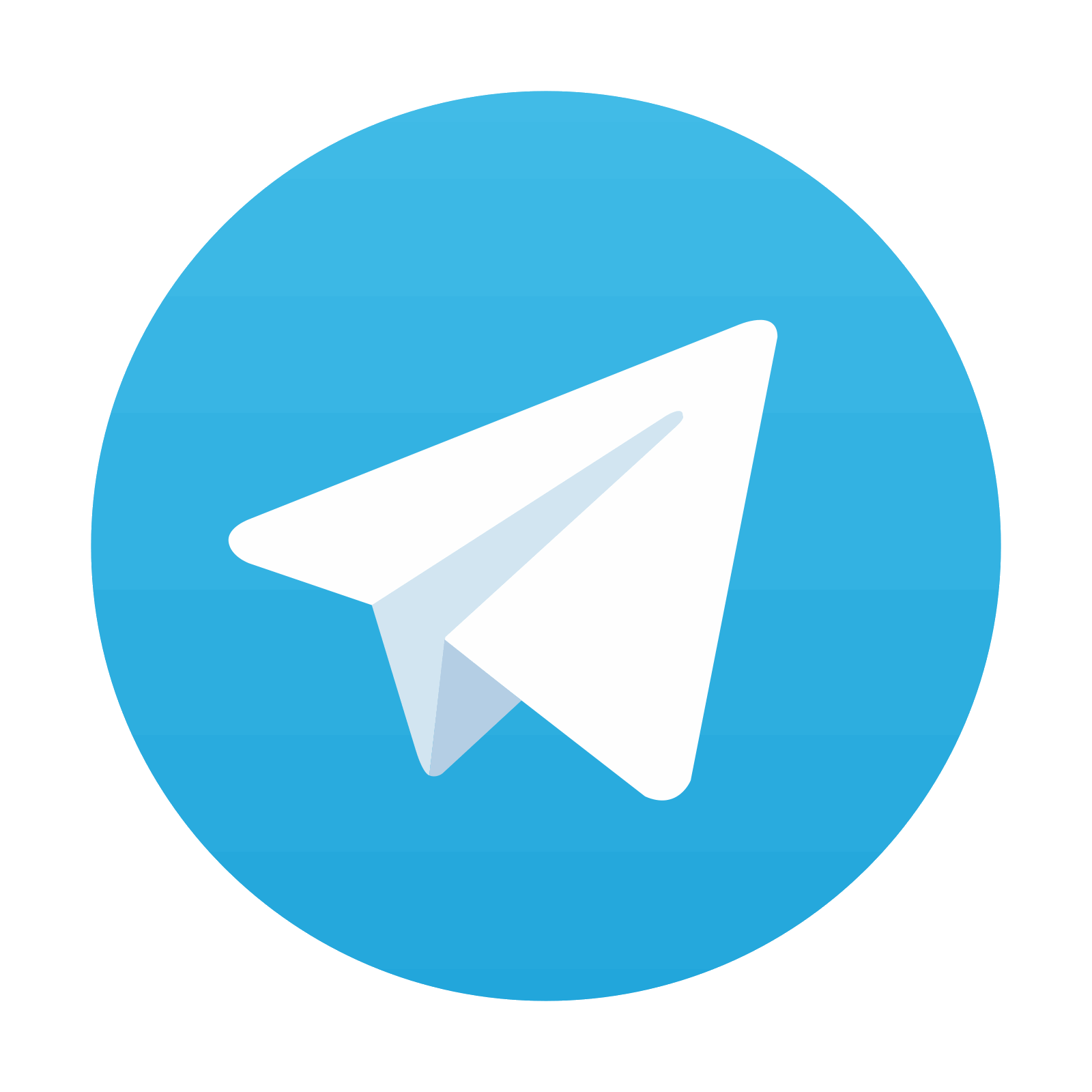
Stay updated, free articles. Join our Telegram channel
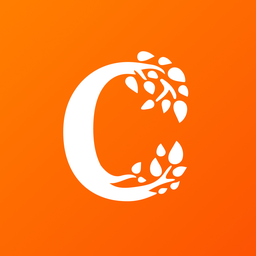
Full access? Get Clinical Tree
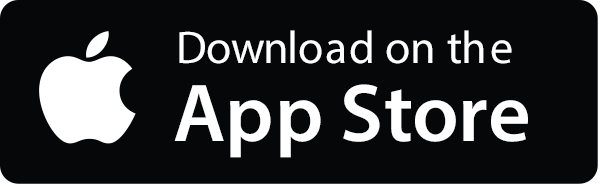
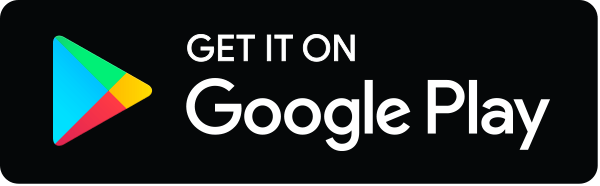