Introduction
Three-dimensional (3D) printing in medicine has gained increasing popularity over the past several years. In nuclear medicine and radiation therapy, where one of the main goals is to obtain quality images while keeping radiation exposure to a minimum, 3D printing is an ideal technology to help tailor many individual treatments. Additionally, 3D printing may be utilized to optimize radiopharmaceutical chemistry for nuclear medicine applications. This chapter will highlight key applications of 3D printing in nuclear medicine and radiation therapy.
Nuclear Medicine
Nuclear medicine uses radioactive substances (radiotracers, radioactive tracers, radionuclides, or radiopharmaceuticals) to diagnose, treat, and monitor a variety of diseases. A radionuclide is an atom that contains an unstable nucleus. As water runs downhill and wood burns, this nucleus will inevitably perform a rearrangement on its path to stability or, in other words, a lower energy state. As postulated by Albert Einstein in his famous E = mc 2 equation, where E = energy, m = mass, and c = the speed of light, energy needs to be conserved; hence, this nuclear rearrangement will produce a release of pure energy, mass, or more often both. Unlike common chemical reactions, nuclear rearrangement is independent of temperature, solvent, and pressure, and will undergo following a very predictable path regardless of the surrounding conditions. In fact, this rearrangement is so predictable that is used as a standard for timekeeping in the form of the atomic clock. These consistent emissions of mass and/or energy interact with surrounding matter and can be detected with proper instruments. Such principles form the base for the nuclear medicine applications discussed in this chapter.
Nuclear emissions may be used for different purposes depending on their nature. Energy emissions in the form of gamma packets (or quantum) are normally used for diagnosis, prognosis, therapy monitoring, and imaging (molecular imaging or MI), as well as internal and external radiation seeds and beams for treatment. Particulate emissions in the form of alpha (∝, high energy 4 He 2+ nuclides) and beta (β, high energy e − ) particles are used for internal radiation treatment (often called targeted radiotherapy or TRT). The different nuclear emissions can be found in Table 12.1 . However, these emitters are often not suitable tools to diagnose nor treat anything on their own, and they must be consequently functionalized to increase their specificity and perform more as radiolabeled pharmaceuticals (or radiopharmaceuticals) rather than just radioisotopes. As Pharmacy is the art, practice, or profession of preparing, preserving, compounding, and dispensing medical drugs, Radiopharmacy is the same but for radiolabeled drugs use in nuclear medicine.
Decay Mode | Emission Type | Composition |
---|---|---|
Alpha decay | α | Helium nucleus, 4 He 2+ |
Beta decay | β – | Electrons, e − |
Positron decay | β + | Antimatter electrons, e + |
Isomeric transition | γ | Pure energy, gamma, high energy light |
From Table 12.1 , it can easily be deducted that most diagnostic radiopharmaceuticals will use isomeric transition radioisotopes to trace the compound behavior and produce medical images. Subsequently, it is also easy to deduct that alpha and beta decays will have the greatest interaction with surrounding matter and should therefore be used for TRT. It is intriguing though and hard to imagine what a “positron” is or what antimatter looks like. The hard truth is we do not know for sure. These antimatter particles last for tiny fractions of a second and are hard to study due to their immediate annihilation with regular matter producing energy. Regardless of our ignorance, we know they exist because the product of such annihilation produces two gamma quanta emitted at almost exactly 180 degrees. The cumuli of these straight angled simultaneous isotropic emissions make these radioisotopes of particular interest for what is called positron emission tomography (PET), the most recent nuclear medicine imaging technique, capable of among other things, measuring noninvasively the metabolic activity of cells in body tissues. PET allows the detection and quantification of the origin of these emissions with the highest degree of accuracy and sensitivity known to date. Common radioisotopes used in PET imaging are shown in Table 12.2 .
Tracer Isotope | Half-Life | Mean Energy (MeV) | Maximum Energy (MeV) | Medical Applications |
---|---|---|---|---|
11 C | 20.4 min | 0.39 | 0.96 | Small molecules, brain imaging |
13 N | 10 min | 0.50 | 1.20 | 13 NH 3 |
15 O | 2 min | 0.72 | 1.74 | H 2 15 O |
18 F | 109 min | 0.25 | 0.63 | Small molecules, brain imaging |
64 Cu | 12.7 h | 0.28 | 0.65 | Medium-sized molecule labeling |
68 Ga | 68 min | 0.89 | 1.92 | Small molecules |
89 Zr | 78.4 h | 0.40 | 0.90 | Protein and antibody labeling |
124 I | 4.2 days | ≈0.7 | 1.532 (11%) and 2.435 (11%) | Protein and antibody labeling |
The traditional medical imaging method utilized in nuclear medicine is called single photon emission computed tomography (SPECT) and is performed using radiopharmaceuticals labeled with pure gamma emitters ( Table 12.3 ). This technique relies on the cumulative detection of single gamma photons emitted by disintegrating nuclei given a certain, predetermined spatial orientation. Planar scintigraphy is the simplest application for single photon emission, and normally produces two 2D images obtained as “anterior” and “posterior” poses. These poses are obtained by rotating the camera around the patient capturing the emitted gamma rays. In contrast SPECT, fundamentally a 3D imaging technique, uses a rotating camera system to obtain many projections and performs tomographic reconstruction to produce imaging slices within the body (similar to CT).
Tracer Isotope | Half-Life | γ Energy (keV) | Medical Applications |
---|---|---|---|
67 Ga | 3.26 days | 93 (40%), 184 (24%), 296 (22%), 388 (7%) | Imaging/blood flow, extravasation, infection. E.g., [ 67 Ga]Ga-Citrate |
99m Tc | 6.02 h | 140.5 (89%), 18.4 (4.0%), 18.3 (2.1%) | 90% of all nuclear medicine apps. E.g., [ 99m Tc] myocardial purfusion imaging test (MIBI) |
111 In | 2.8 days | 171.3 (91%), 245.4 (94%) | White blood cell labeling, octreotides |
201 Tl | 3.04 days | 70.8 (46.5%), 68.9 (27.4%), 80.3 (20.5%), 167.4 (10.0%) 135.3 (2.7%) | Cardiac |
Other gamma emitting radionuclides ( 137 Cs (t 1/2 = 30.2 years) and 60 Co (t 1/2 = 5.27 years)) can be used for external beam radiation therapy because their high energy emissions can penetrate deep into human tissue. These γ-beams can be properly collimated and directed specifically to the treatment site with great accuracy. These, however, cannot be used to produce radiopharmaceuticals due to their long half-life. In contrast, internal radiation therapy or targeted radiotherapy is a treatment in which a source of radiation (normally an alpha or beta emitter) is put inside of the body ( Table 12.4 ). The radiation source can be solid (brachytherapy) or liquid (systemic therapy). In brachytherapy, seeds, ribbons, or capsules with a radiation source are placed in or near a tumor and these solid particles give off radiation (typically gamma). In systemic therapy, the treatment is given orally or intravenously and travels through the blood or tissues, seeking out and killing cancer cells.
Tracer Isotope | Half-Life | Energy (Particle) | Medical Applications |
---|---|---|---|
90 Y | 2.7 days | 2.28 MeV (β − ) | Liver, neuroendocrine |
177 Lu | 6.65 days | 0.497 MeV (β − ) | Prostate, neuroendocrine |
188 Re | 16.9 h | 2.12 MeV (β − ) | Bone, radioimmunotherapy |
223 Ra | 11.4 days | Multiple α and β − decays | Bone, prostate |
225 Ac | 10.0 days | Multiple α and β − decays | Prostate, brain |
All these radionuclides can seldom be injected as ions without chemical modification, and they often need to be administered as part of bigger construct called Radiopharmaceuticals . These cannot be produced and stored for administration because of decay and the very same characteristic for which they are used: ionizing radiation and radiolysis. Therefore, radiopharmaceuticals need to be produced fresh before every administration, making this process costly and demanding of well-trained personnel. To avoid decay and radiolysis, adhere to good manufacturing pharmaceuticals guidelines, reduce costs, reduce personnel exposure to radiation, and assure drug quality and reproducibility these processes need to be automated. Several commercial automated units are available in the market, but their costs rise well above $100,000.00, and they are not optimized for a particular synthesis. These units are what we call a “jack of all trades and master of none.” To address the need to automate novel, specific reactions while lowering costs, new design and fabrication techniques must be introduced, and such is the case of 3D printing.
3D Printing Techniques to Optimize Radiopharmaceutical Chemistry
The diffusion and assimilation of 3D printing technologies exemplifies ease-of-use, affordability, and versatility. Since the number of radiopharmaceuticals is bound to increase with the discovery of new molecular targets, the development of novel ligands, the availability of previously rare positron emitting radionuclides, as well as the inevitable increase of the patient population, new synthetic and reproducible techniques have to be introduced to accommodate dissimilar syntheses. The reactions that bind these radionuclides to their relevant ligands must be completed in less than one physical half-life of the radionuclide. These reactions also need to be performed with high fidelity while satisfying the regulatory concerns of administering injectable radioactive compounds to patients. These 3D printing technologies can be easily combined with recent advanced in robotics components and the reduction in their prices, to create a reliable automated synthesis unit (ASU) at a fraction of the cost (1/20th) of the commercially available ASUs.
The low cost of the ASUs achieved by 3D printing bring along a novel concept in radiopharmaceutical chemistry: “Do not accommodate the synthesis to the existing ASU, but create the ASU to fit the optimized synthesis.” Such a revolutionary concept allows for true good manufacturing practices in drug production, since the process is tailored to assure quality instead of trying to build quality into existing processes. This quality is achieved by maximizing radiochemical yields and final radiochemical purities of the produced drug products by design, and not as a result of a reaction adaptation to unfit automation.
Example 1: [ 11 C] Fatty Acid ASU
Fatty acids labeled with 11 C allow clinically relevant metabolic processes to be traced “in vivo.” However, 11 C is a challenging isotope to work with since its half-life is only 20.2 min. To achieve injectable amounts of compounds post labeling and quality control, it is necessary to start with high amounts of activity (1–5 curie), which cannot be manipulated by operators. Hence, automation is a must. Fatty acids are often produced by bubbling traces of 11 CO 2 carried by a stream of N 2 , He, or Ar. The reasons why such methods are conceptually unfit for radiochemistry is a discussion for a different book; however, efforts to change this practice have been performed and superior yields, superior purity, and decreased synthesis times have been achieved. The major challenge for such alternative methods is the fact that such processes have never been automated, and there is not a single commercial system to accommodate them.
The combination of 3D printing, high torque servos, linear motors, and a controlling chip to which sequences can be saved and executed on demand yielded a breakthrough in automation for radiopharmaceutical production ( Fig. 12.1 ). The ASU improved the manual syntheses by reducing synthesis time from 12 to 8 min and produced consistent decay corrected radiochemical yields. Furthermore, the ASU was capable of producing not one but three tracers of clinical interest which included [ 11 C]acetate, [ 11 C]palmitate, and [ 11 C]propionate at higher than previously reported yield, in only 8–10 min.
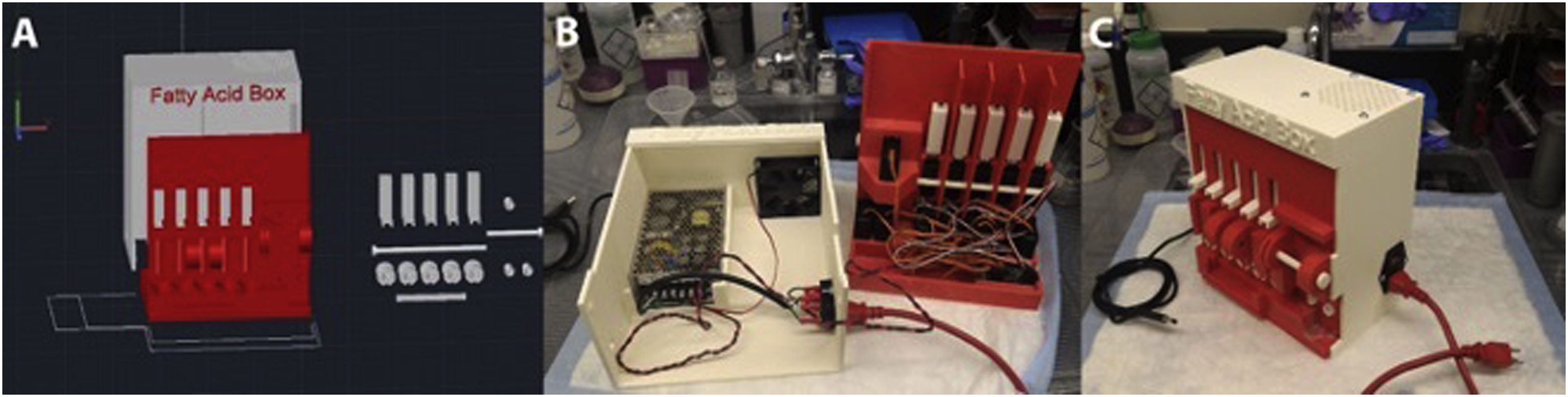
Example 2: [ 18 F] Dual Heater ASU
The half-life of 18 F (t 1/2 = 109 min) is more than three times larger than that of [ 11 C]. This allows to accommodate longer synthesis times and more complex, multistep reactions. However, almost none of the commercially available ASUs are fit to perform complicated reactions, and the few that are cost more than US $150,000. Such costs are prohibitive during the testing of compounds for early agency approval for most researchers, therefore an alternative was needed. Once again, 3D printing combined with robotics solved this problem, specifically for the multistep radiosynthesis of the prostate-specific membrane antigen (PSMA) [ 18 F]RPS-040 for preclinical PET imaging which involved two separate reactions and a distillation. The total cost for developing this using 3D printing and robotics was US $7,000, approximately 5% of the commercially available ASU.
In order to perform the first automated synthesis of [ 18 F]RPS-040, an ASU was designed and assembled. To accommodate the high temperatures needed during this synthesis, the porcelain reactors’ housing was 3D printed with clay using material extrusion technology. The 3D printing process of clay was optimized to account for shrinking in the kiln, and it resulted in a perfect 3D printed porcelain part with less than 1 mm error. A pierce element was used in the second reactor to cool it down to 0°C for the distillation of the intermediate and heat it up to 100°C for the following reaction steps ( Fig. 12.2 ). The synthesis time was reduced from approximately 2 h manually to 65 min, producing a product needing only a final High-Performance liquid chromatography (HPLC) purification and reformulation for injection. Such ASU was unprecedented, and the complicated, multistep synthesis was automated, optimized, and tested without a single mechanical failure in more than 20 trials.
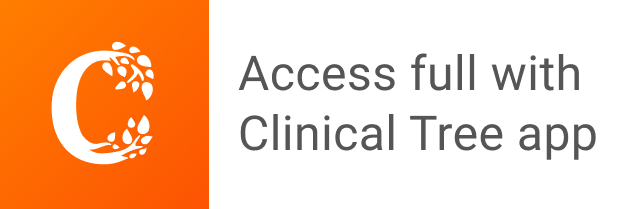