Fig. 48.1
(a) Digital subtraction angiography demonstrating the three main components of an AVM: arterial feeding pedicles (small arrow), nidus (circle), draining vein (thick arrow) into the superior sagittal sinus. (b) Digital subtraction angiography using iFlow analysis (Siemens, software version 20) of an AVM; arterial (red) and venous (blue) phases. Notice that the superior sagittal sinus (SSS) is yellow rather than blue, depicting early venous drainage from the AVM. Additionally, cortical veins not associated with the AVM are blue (small arrows) suggesting that the AVM does not drain through these veins. (c) Intraoperative visualization of well-demarcated flow (arrow) between arterial (red) and venous (blue) blood in an arterialized cortical vein associated with an AVM
Natural History of Arteriovenous Malformations
The prevalence of AVMs in the general population is less than 1 % [8–11]. AVMs can manifest with seizures, headache, cognitive deficit, pulsatile tinnitus, or other focal neurological deficits. The most common presentation is intracranial hemorrhage associated with a 50 % morbidity and mortality [12, 13]. AVMs are the most common cause of intracranial hemorrhage in children and young adults [13, 14]. The risk of hemorrhage in unruptured AVMs is estimated to be about 2–4 % annually [9, 11, 15–22] but is cumulative such that patients diagnosed at a younger age are at a higher risk of bleeding during the course of their lifetime. After the initial hemorrhage, the risk of another bleed is 18 % in the first 6 months and returns to baseline by 3–5 years [15, 20, 22].
The abnormally high flow and pressure within the AVM contribute to the bleeding and can also result in aneurysm development. AVM-associated aneurysms further increase the annual bleeding risk to 41 % [12, 15, 23]. These aneurysms are likely the result of abnormal shear stress on the arterial walls creating further weakening with aneurysm formation. Venous aneurysms, or varices, form in the venous system and can bleed, particularly when the vein distal to the varix is stenotic, further increasing the pressure within the venous aneurysm.
Arterial aneurysms are classified by location relative to the AVM: (1) intranidal, (2) extranidal, and (3) flow related (Fig. 48.2). The first two types are directly associated with the vasculature of the AVM, whereas the flow-related aneurysms are located elsewhere on the circle of Willis (COW) [24]. Intranidal aneurysms were reported in 13.6 % of AVMs, extranidal aneurysms in 10.9 % of AVMs, with the latter being flow-related 72 % of the time and in the COW 27 % of the time [24]. The etiology of the AVM-associated aneurysms seems related to the abnormally high-flow within the AVM vasculature, but it is less clear how the distant ones form. Though speculative, distant aneurysms may result from structural wall defects that arise either from congenital or acquired etiologies that increase the susceptibility of these vessels to aneurysm formation.
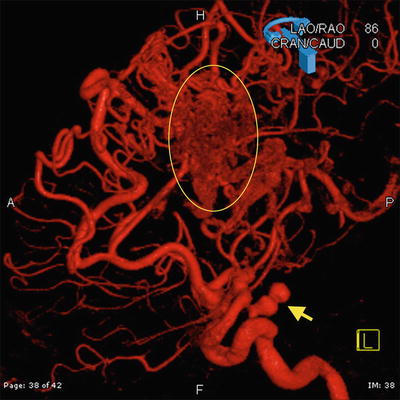
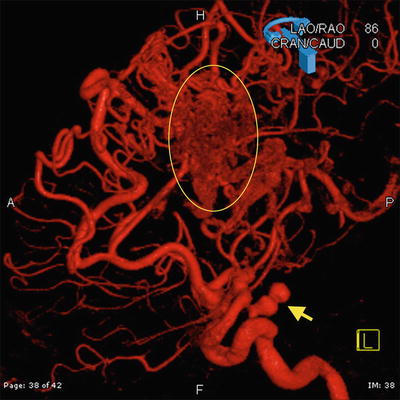
Fig. 48.2
3D digital subtraction angiogram demonstrating AVM nidus (circle) and an extranidal AVM-associated multilobulated aneurysm (arrow)
Clinical Presentation and Initial Assessment
Patients with unruptured AVMs most often present with chronic headaches or seizures, while ruptured ones present with severe acute headache and neurological deficits [12, 21, 25]. Risk factors for AVM bleeding are often controversial with conflicting reports in the literature. However, some of the more accepted risk factors include prior hemorrhage, associated aneurysm, venous varix, and venous outflow stenosis, while nidus size remains controversial [15, 16, 23, 26].
In the case of patients who present with ruptured AVMs, initial assessment can be artificially divided into two categories: hemorrhage with and without symptomatic mass effect. Clearly, patients with AVM bleeding significant enough to cause severe neurological deficit from mass effect should be considered for operative intervention to evacuate hematoma and resect the AVM. However, the extent of surgical resection in these emergent situations is often decided intraoperatively depending on the size and location of the lesion. For example, the entire AVM may not be removed at the time of clot evacuation if it is located in eloquent regions because it could exacerbate neurological deficits. In this situation, it would be better to evacuate clot leaving the AVM behind to limit further neurological deficits and prevent profuse bleeding intraoperatively.
In situations where there is no symptomatic mass effect, timing of treatment becomes more complex. Some neurosurgeons prefer to delay treatment until the clot retracts, cerebral edema resolves, and elevated intracranial pressure subsides, using only aggressive medical management as needed [27]. Acute surgical intervention in this setting can make treatment more precarious and potentially increase the risk of dangerously elevating intracranial pressure. For example, if preoperative embolization is being considered in the acute phase, a second bleed, and an ischemic event or redirection of the flow in the AVM from vascular occlusion, could result in additional edema and mass effect, forcing surgical intervention. Similarly, preoperative angiography in the setting of brain shift and edema may not allow full and accurate visualization of the AVM angioarchitecture, making complete resection less likely and more difficult. Delaying intervention until the hematoma, edema, and elevated intracranial pressure resolve followed by elective noninvasive functional brain mapping and angiography will enable the neurosurgeon to operate more safely with better outcomes than in the acute scenario.
Imaging Modalities for Presurgical Planning
In order to appropriately choose candidates for surgical resection, imaging modalities assessing the angioarchitecture of the AVM, surrounding cortical eloquence, and anatomy of the white matter tracks can be helpful during preoperative planning and discussions with the patient about surgical risks. Not all patients with AVMs are surgical candidates particularly with the advent of endovascular embolization and SRS [6]. Here, we will discuss the utility of the Spetzler-Martin grade and imaging modalities during preoperative treatment planning.
AVM Surgical Grading Systems
The AVM size (<3 cm, 3–6 cm, >6 cm), location (eloquent or non-eloquent), and venous drainage pattern (deep or superficial) were found to be important AVM characteristics when determining surgical risk and predicting postoperative neurological outcomes [28]. The Spetzler-Martin grading system (SMG) was developed using size, location, and venous drainage patterns to assign a numeric score (1–5) with the higher number predicting higher surgical risk with worse neurological outcomes (Table 48.1); an SMG of 6 has been used to imply an “unresectable” AVM. This system was validated and predicts that an SMG of “1” has a 0 % risk of deficit from surgical resection, while an SMG of “5” has a 31 % risk. The authors further divided surgical risk into “major” and “minor” neurological deficits (Table 48.2). Though the SMG has been very useful to many surgeons planning for AVM resection, it does have pitfalls. For example, surgical outcomes associated with the SMG will be different for individual surgeons depending upon their training, technical abilities, case volume, and experience. Additionally, interobserver variability for the SMG was 28 %, with venous drainage having the least interobserver variability and nidus size having the greatest [29].
Grading feature | Points assigned |
---|---|
Size of nidus | |
Small (<3 cm) | 1 |
Medium (3–6 cm) | 2 |
Large (>6 cm) | 3 |
Eloquence of adjacent brain | |
Non-eloquent | 0 |
Eloquent | 1 |
Pattern of venous drainage | |
Superficial | 0 |
Deep | 1 |
Grade | No deficit (%) | Minor deficit (%) | Major deficit (%) |
---|---|---|---|
1 | 100 | 0 | 0 |
2 | 95 | 5 | 0 |
3 | 84 | 12 | 4 |
4 | 73 | 20 | 7 |
5 | 69 | 19 | 12 |
A modification of the SMG was subsequently described where age, rupture status, and nidus diffuseness were considered important characteristics for assessing surgical outcome. This modification was published as the “supplementary grading system” (SGS) developed by reviewing 300 patients who underwent microsurgical AVM resection and determining their modified Rankin score, SMG, and SGS (Table 48.3) [30, 31]. The predictive accuracy of the SGS for postsurgical neurological deficits was higher (0.73) than the SMG (0.66). However, the SGS is intended to be an adjunct to the SMS and not a substitute. Therefore, the modification includes adding the SMG and SGS scores to obtain a combined score ranging from 0 to 10. Using this scoring system, a grade of 1–3 predicts low surgical risk, 4–6 moderate risk, and 7–10 high risk, for surgical AVM resection.
Grading feature | Points assigned |
---|---|
Age | |
<20 years | 1 |
20–40 years | 2 |
>40 years | 3 |
Unruptured presentation | |
No | 0 |
Yes | 1 |
Diffuse | |
No | 0 |
Yes | 1 |
A three-tier classification system was recently described in order to guide AVM management based on the SMG [32]. AVMs were classified into “A” through “C” based on SMG with recommended management options according to the classification (Table 48.4). The recommendations based upon this classification system were Class “A” (SMG 1, 2), surgical resection; Class “B” (SMG 3), multimodality treatment; and Class “C” (SMG 4, 5), no treatment.
Class | Spetzler-Martin grade (SMG) | Treatment |
---|---|---|
A | 1 and 2 | Surgical resection |
B | 3 | Multimodality |
C | 4 and 5 | No treatment |
Imaging Studies
Various imaging modalities can be used to depict the anatomical features of an AVM, including computed tomography angiography (CTA), magnetic resonance angiography (MRA), and magnetic resonance venography (MRV) (Fig. 48.3). However, only catheter-based digital subtraction angiography (DSA) can demonstrate the anatomical and hemodynamic features of the AVM. Differentiating between arterial feeding pedicles and draining veins can be challenging on the static imaging such as CTA and MRA (Fig. 48.3). Additionally, clearly identifying the extent of the AVM nidus on MRA and CTA can also be challenging at times, particularly with diffuse lesions. Static images, however, unlike DSA, depict the brain tissue relative to the AVM and are therefore very important for determining the location of the AVM within the cerebral hemispheres. These static imaging modalities are particularly useful for determining AVM proximity to eloquent cortical and subcortical areas. Newer static imaging modalities such as functional MRI (fMRI) and diffusion tensor imaging (DTI) can be particularly useful for preoperative planning.
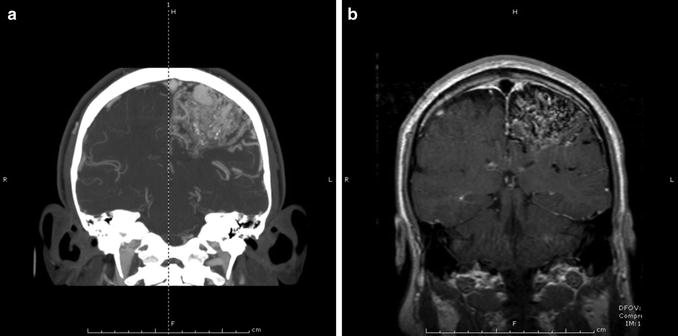
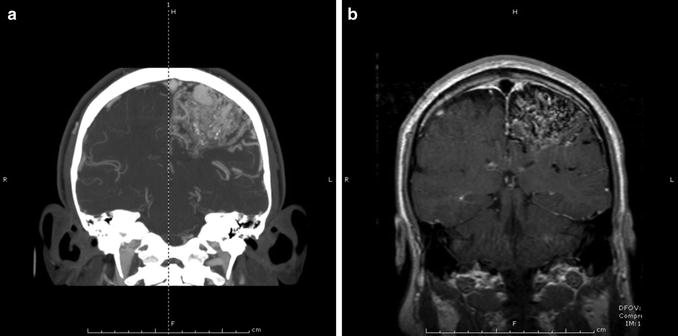
Fig. 48.3
Coronal section of a CTA (a) and MRA (b) depicting the three main architectural components of an AVM: arterial feeding pedicles, nidus, and venous drainage. These static images make it difficult to distinguish between arterial and venous structures and determining the precise boundaries of the AVM nidus
Functional MRI
The emergence of FMRI in the 1990s and its continued development enable clinicians to identify eloquent cortical areas using real-time noninvasive mapping techniques. The patient is presented with a series of tasks during an MRI and the cortex can be mapped based on changes in blood flow and oxygen delivery, making this a functional and physiological test for detection of eloquent brain tissue. The proximity of eloquent areas relative to the AVM can then be viewed when planning the surgical approach and assessing surgical risk with the patient (Fig. 48.4). The combined use of noninvasive static imaging (MRA, fMRI, CTA) and minimally invasive dynamic imaging (DSA) allows the neurosurgeon to apply the SMG in a more precise manner when considering potential treatment options [33]. For example, an AVM clearly located in the eloquent cortex or deep white matter structures would likely result in an unacceptably high surgical risk, making embolization or SRS a safer option. fMRI is also helpful with AVM treatment planning because a small percentage of individuals exhibit cortical rearrangement, such that functionality is not where one would typically expect. These functional anomalies could have important implications for surgical planning when they are identified ahead of time and avoid unexpected poor outcomes from neurological deficits (Fig. 48.5).
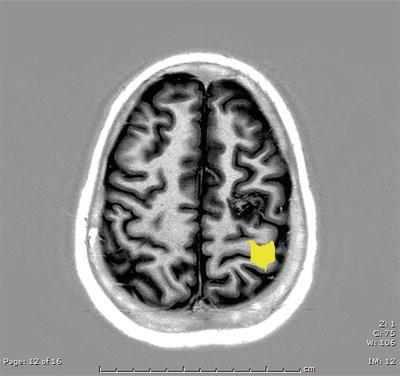
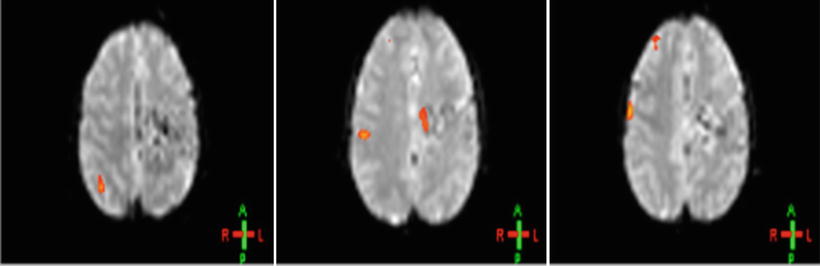
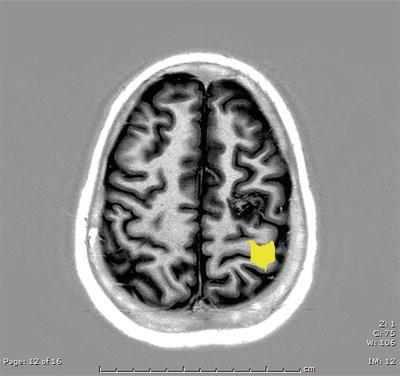
Fig. 48.4
fMRI depicting vascular flow voids from the AVM in the left frontal lobe anterior to the right-hand motor cortex (yellow blob)
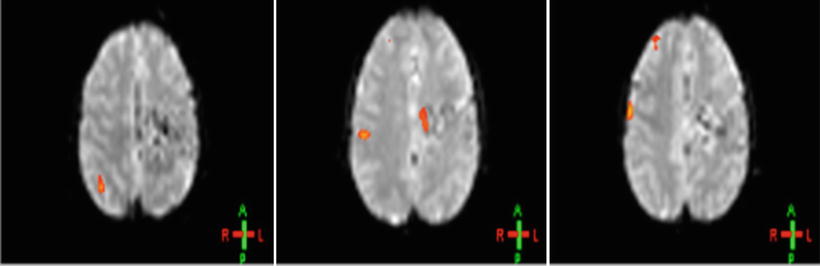
Fig. 48.5
fMRI demonstrating cortical rearrangement in a left-handed individual who was asked to tap his right fingers. Notice bilateral cortical involvement (red blobs) with the left cortical activity lying deep, medial to the AVM flow voids
Diffusion Tensor Imaging
DTI is a relatively new form of MRI technology that uses water diffusion in the brain tissue to map the white matter tracks (tractography) throughout the hemispheres (Fig. 48.6). The result is a color-coded map of the white matter tracts that can be viewed relative to the location of the AVM. White matter tracts originating from eloquent structures passing through the AVM nidus could be an important deterrent to surgical resection. For example, surgical resection of an AVM nidus adjacent to the white matter tracts connecting Broca and Wernicke areas may result in a conductive aphasia from disconnection of these fibers. Another example would be an AVM in Meyer’s loop resulting in visual field deficits after resection. In this way, DTI tractography can be an important tool for presurgical risk assessment and may guide the decision towards a minimally invasive therapy that is less likely to injure critical brain structures.
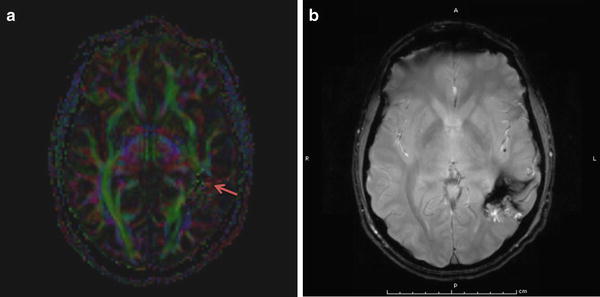
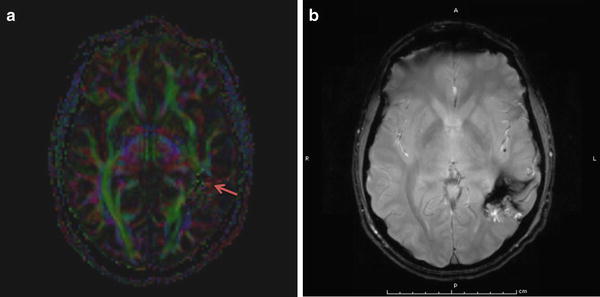
Fig. 48.6
DTI tractography demonstrating white matter tracks surrounding the AVM (a) with corresponding MRI (b) allowing localization of the AVM relative to critical brain structures. Depicted here is a left parieto-occipital AVM in a patient who presented with receptive aphasia, visual field cut, and dyslexia after hemorrhage. The arrow in (a) points to a disruption in the white matter tracts seen as a black void
Presurgical Embolization
The first reported AVM embolization was performed by Drs. Luessenhop and Spence in 1960. They reported near-total angiographic occlusion of an AVM using methyl methacrylate embospheres [34]. Since that time many different materials have been developed for embolization, including calibrated particles, silk thread, absolute ethyl alcohol (EtOH), and polyvinyl alcohol (PVA). Liquid embolic agents have been developed with the advantage over particles because they form a vessel cast resulting in better occlusion and are less likely to migrate into the venous system when there is a high-flow fistulae.
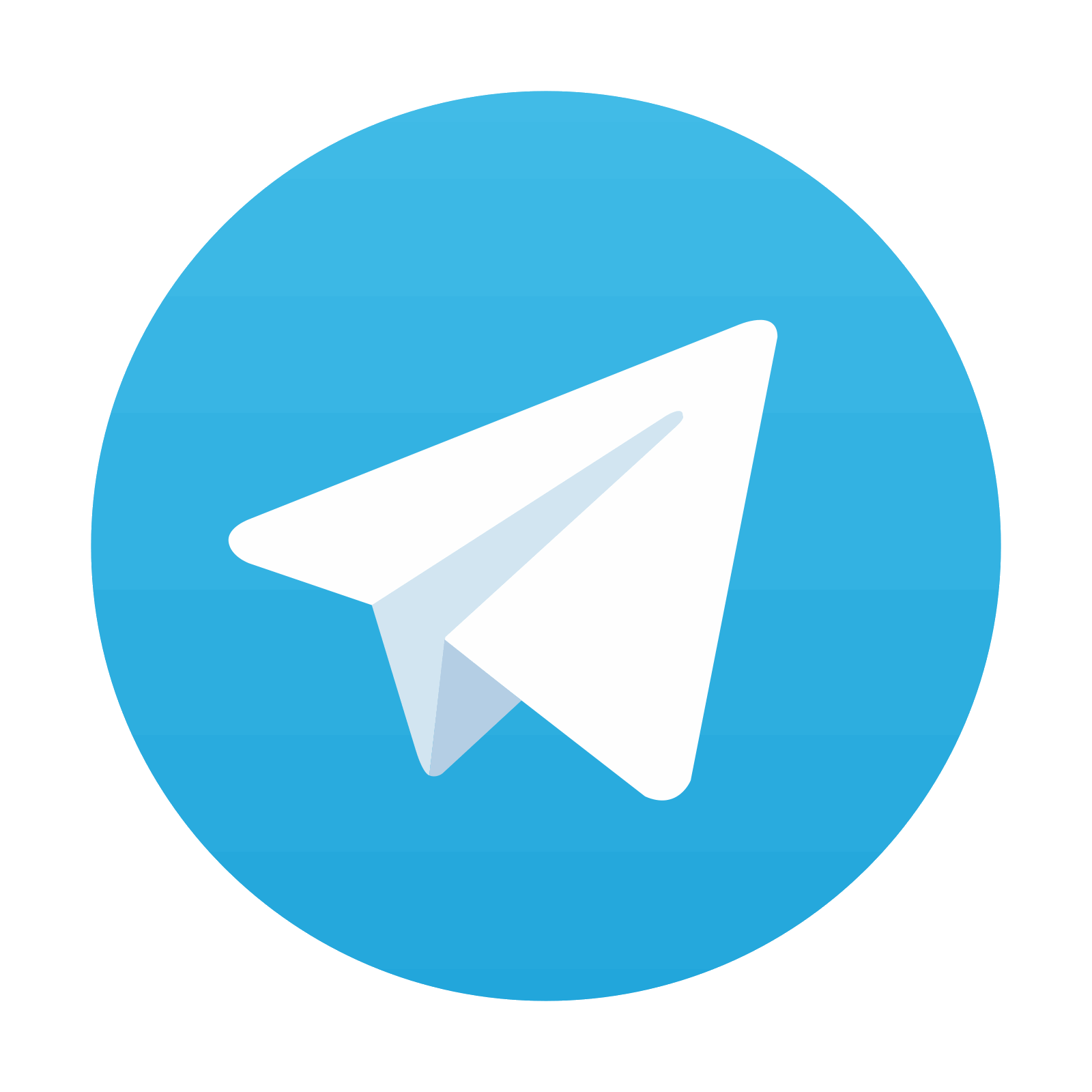
Stay updated, free articles. Join our Telegram channel
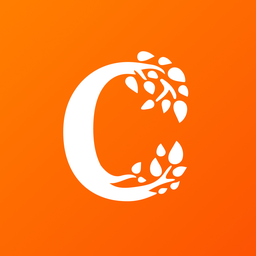
Full access? Get Clinical Tree
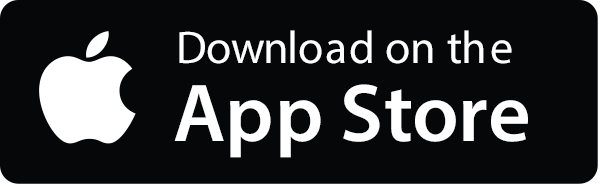
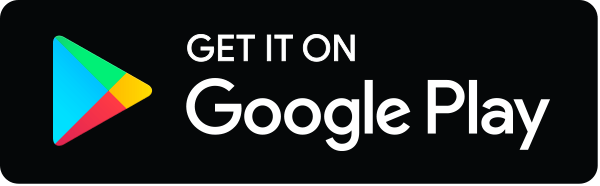