Abstract
Over the past decade, significant strides have been made in advanced non-invasive cardiac imaging. Technological advances in both hardware and software applications have made direct visualization of atherosclerotic plaque a reality. These advances have come at a crucial time, and help to fill a gap in contemporary diagnostic modalities, which have additionally translated to refining cardiovascular prognosis. In this chapter, the utility and application of arterial ultrasound, coronary computed tomography, cardiac magnetic resonance imaging, single-photon emission computed tomography, and positron emission tomography will be discussed.
Keywords
Atherosclerosis, Plaque, Inflammation, Positron emission tomography, Magnetic resonance imaging, Carotid ultrasound, Cardiac computed tomography
8.1
Introduction
Atherosclerosis is a chronic inflammatory disease [ ]. Although great strides have been made in the diagnosis and management of atherosclerotic cardiovascular disease (CVD), overall mortality due to underlying atherosclerosis remains a leading cause of death in industrialized countries [ , ]. For example, more than one-third of all deaths in the United States are attributed to CVD, including atherosclerotic coronary disease and stroke [ ]. By 2030, it is projected that more than 148 million of the US population would have heart disease. Given the gravity of this situation, it is of paramount importance to improve cardiac risk assessment in order to identify at-risk individuals. Significant advances have been made over the past decade in noninvasive cardiac imaging, permitting earlier detection of vascular atherosclerotic disease beyond conventional risk calculator assessments. The ability to directly visualize atherosclerotic plaque has spawned further efforts to refine imaging-based detection of plaque with ultrasound (US), computed tomography (CT), magnetic resonance imaging (MRI), single-photon emission computed tomography (SPECT), and positron emission tomography (PET). This chapter will focus on the utility and applications of noninvasive imaging modalities—US, CT, MRI, SPECT, and PET—and their individual, yet complementary roles in the detection and characterization of atherosclerotic plaque.
It is well documented that atherosclerotic plaque rupture is the underlying pathophysiologic mechanism governing the majority of CVD complications, including myocardial infarction (MI), stroke, and sudden cardiac death [ ]. Despite contemporary guideline-based management for at-risk and established CAD patients, cardiovascular events persist at an alarming rate. This risk is thought to be due to unabated systemic inflammation [ ]. Inflammation is at the crossroads of atherosclerotic plaque initiation, progression, and complications from atherothrombosis [ ]. There are many notable steps in the inflammatory cascade that lends to atherogenesis: endothelial cell damage, up-regulation of endothelium adhesion molecules, accumulation of oxidized lipoproteins, monocyte recruitment and subsequent foam cell formation, angiogenesis, microcalcifications, and apoptosis. Unperturbed, these highly coordinated molecular and cellular events result in plaque destabilization. This end event is mediated by activated inflammatory cells, notably macrophages, which release degradative enzymes and confer an increased risk for plaque rupture. In addition to inflammation, other plaque properties such as presence of thin-cap fibroatheroma and lipid-rich necrotic core define an inflamed or high-risk plaque, which are the culprit lesions responsible for vascular atherothrombosis and adverse CV events [ ].
Accordingly, early detection of both atherosclerotic disease and high-risk plaques is crucial to abating the total burden of CVD and its potential clinical complications [ , ]. In this regard, the use of noninvasive imaging techniques offer substantial advantage over standard CV risk calculators or invasive modalities, by affording the assessment of both anatomical and functional atherosclerosis parameters before the onset of clinical manifestations.
8.2
Non-invasive imaging of atherosclerosis
8.2.1
Carotid artery ultrasound
Carotid ultrasound allows visualization of the carotid arteries with an axial and lateral resolution of 0.044 and 0.25 mm, respectively. Intima-media thickness (IMT) is represented by the measured longitudinal distance between: (1) lumen-to-intima, and (2) media-to-adventitia boundaries [ ]. The identification of a carotid atherosclerotic plaque is defined as focal IMT thickness of 50% or > 0.5 mm relative to the adjacent arterial wall, or alternatively, an absolute IMT > 1.5 mm [ ]. Carotid IMT (CIMT) using ultrasound, with standardized protocols and excellent sonographers and readers, lends to highly reproducible IMT measurements [ ].
Another form of carotid ultrasound involves the use of injected contrast, intravascular microbubbles, which permit the visualization of micro- and macro-vasculature, and notably, intraplaque neovascularization [ ], as validated by histopathologic exam. Neovascularization occurs early in atherogenesis, whereby the formation of leaky micro-vessels within plaque pose an increased risk for hemorrhage and potentiation of inflammation, leading to plaque instability [ ] ( Figure 8.1 ). As such, detection of neovascularization by contrast-enhanced ultrasound (CEUS) is a potential marker of high-risk atheromatous lesions. The diagnostic yield of CEUS has been explored in a few studies. In one such study, CEUS with perflubutane was used in 50 patients prior to carotid endarterectomy. We measured enhanced intensity and assessed the correlation between contrast effect and histopathology, comparing symptomatic and asymptomatic plaques. In both symptomatic and asymptomatic plaques, the correlation between CEUS based intensity in the plaque shoulder was associated with neovessel density ( P < 0.01; ρ = 0.43). Furthermore, CEUS intensity of the plaque shoulder was greater in ruptured plaques than those without ( P < 0.05), and in symptomatic versus asymptomatic plaques ( P < 0.01). These compelling results warrant larger longitudinal studies to explore the potential prognostic utility of CEUS.
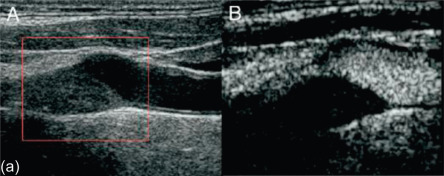
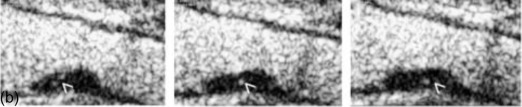
Another advance in ultrasound plaque imaging involves the use of 3-dimensional (3D) ultrasound imaging, which builds upon many of the core principles of ultrasound imaging. This ultrasound technique enables not only visualization of carotid plaque, but more accurately quantifies plaque size and vessel volume. Plaque volume, as assessed with 3D ultrasound, has been shown to be a more robust metric than CIMT alone; hence, a highly sensitive means to detect plaque progression, by over two orders of magnitude more than IMT [ ]. Since atherosclerotic plaque increases in size faster longitudinally than it thickens, employing 3D ultrasound allows for smaller sample sizes in the serial assessment of an intervention on plaque progression [ ]. Thus, the role of 3D ultrasound plaque imaging in drug trials may prove to be cost-effective and lead to more testing of novel pharmacotherapeutics. Moreover, 3D ultrasound holds predictive and incremental value to coronary artery calcium scoring (CACS) [ ], a well-accepted biomarker of CAD that carries prognostic value. The ability of 3D-CIMT to predict subsequent CVD events is actively being studied in the BioImage study (clinicaltrials.gov NCT00738725) and the ability to detect high-risk plaque features in the Canadian Atherosclerosis Imaging Network (NCT01456403).
8.2.1.1
CIMT and cardiovascular risk
The value of CIMT measures is buttressed by a strong association between increased CIMT and major traditional cardiac risk factors [ , ]. Furthermore, epidemiological studies have demonstrated substantial predictive value of CIMT for future CVD events, independent of well-recognized traditional cardiovascular risk factors [ ]. The net reclassification index (NRI) is significant with the addition of maximum CIMT of the internal carotid artery ( P < 0.001). Additional IMT indices have also been investigated. For example, in the Atherosclerosis Risk in Communities (ARIC) study, the presence of plaque in addition to increased CIMT improved the NRI by 9.9% [ ]. Notably, the presence of hypoechoic plaques (indicative of lipid-rich content), has been shown to be an independent predictor of CV death [ , ].
8.2.1.2
CIMT and clinical trials
CIMT is used to quantify the degree of early atherosclerosis and to monitor the extent of modulation in response to novel therapies, in particular those that target lipids and blood pressure. In one study, a significant reduction in CIMT progression during statin therapy [ ] was demonstrated. Although there was no regression of plaque in a separate study, a greater reduction of IMT progression was observed in response to intensification of statin therapy [ ]. The results of these CIMT based trials are concordant with the results of clinical outcomes in clinical studies [ , ]. Despite these promising results with anti-lipid therapy, these results have not been replicated with anti-hypertensives or non-statin agents [ ]. Additional studies using plaque-imaging ultrasound techniques are necessary to determine the overall utility and prognostic vale of this technology among the landscape of other imaging modalities, to be discussed in this chapter.
Advantages of ultrasound technology, in comparison to CT, MRI, and nuclear imaging, rest in its broader availability and lower cost profile. Importantly, ultrasound-based plaque imaging affords a reproducible measurement that does not involve any radiation exposure. It has demonstrable value in predicting future CV risk, independent of traditional CV risk factors. For all these reasons, plaque imaging by ultrasound (i.e., CIMT, 3D ultrasound, CEUS) is an attractive modality to employ in the risk assessment of early atherosclerosis in patients.
8.3
Computed tomography
8.3.1
Coronary artery calcium scoring
CACS can be performed by noncontrast-enhanced computed tomography [ ]. CACS is a relatively simple technique associated with a low radiation dose in the range of ~ 1 mSv [ ]. The conventional Agatston score is calculated by measuring areas with a CT attenuation value above 130HU (Hounsfield units), multiplying each calcified lesion by a factor related to the maximum attenuation value, and then adding up the per-slice scores to acquire a total calcium score. Alternative measures of coronary calcium, the calcium volume, the calcium mass, or calcium density may be more reproducible or provide incremental prognostic value [ ]. Nevertheless, because of its long existence and familiarity, the Agatston score remains the most frequently reported coronary calcium measure, and correlates well with overall atherosclerotic burden. In the general population, the calcium score increases with age, is higher in men than women, and appears to vary by race.
8.3.1.1
CACS and cardiovascular risk
Multiple studies have investigated the prognostic value of the relationship between CACS and atherosclerosis [ , ]. For example, in one of the largest studies, inclusive of 25,253 asymptomatic middle-age men and women, coronary calcium score predicted all-cause mortality and did so more accurately than traditional CAD risk factors [ ]. In addition to this large population study, in the Multiethnic Study of Atherosclerosis (MESA), increasing CACS portended a higher risk of future major adverse cardiovascular events (MACE). In MESA, 6724 asymptomatic individuals were compared to individuals with zero CACS. The results demonstrated that CACS of 1–100, 101–300 and > 300 are associated with a 3.6-, 7.7- and 9.7-fold increased risk of MACE at 3.8 years, respectively [ ]. These findings are independent of age, gender, or ethnicity [ ]. In addition, recent data from MESA has shown that although higher CACS scores are associated with increased MACE, a lower density of calcified plaque is associated with lower MACE [ ]. Future studies are required to determine the incremental predictive utility of CT densities.
Not only is CACS a robust independent predictor of CV mortality, but the presence of CAC provides incremental value beyond Framingham risk estimates, as well as various biomarkers, for prediction of CV events and mortality [ ]. In a study of 4609 asymptomatic individuals, CAC progression provided incremental value in predicting all-cause mortality over baseline score, time between scans, demographics, and CV risk factors [ ]. Lastly, the detection of CAC has potential clinical benefits by earlier initiation of therapeutic lifestyle changes and/or medical therapy, or patient compliance, although a direct effect of calcium scanning on outcome has not yet been demonstrated [ ]. As it stands, current guidelines consider calcium scanning useful to further risk stratify patients at intermediate risk by conventional risk factors. Cohort studies have demonstrated that a calcium score improves risk estimation in these intermediate risk patients, with potentially more effective allocation of preventive measures.
8.3.1.2
CACS and clinical trials
Over the past two decades, a number of published studies used CACS progression as an endpoint. In one of these studies, a randomized controlled outcome trial [ ], the utility of CACS to identify patients who may derive benefit from medical treatment was studied. This study, the St. Francis Heart Study, comprised 1005 asymptomatic individuals with CACS > 80th percentile that were age- and gender-adjusted. At 4.3 years, treatment with atorvastatin 20 mg/dl reduced low-density lipoprotein cholesterol by 39% ( P < 0.0001), and triglycerides by 11% ( P = 0.02), while reducing clinical endpoints (coronary death, nonfatal MI, coronary revascularization, ischemic stroke, and peripheral arterial surgery) by 30% (6.9% vs. 9.9%, P = 0.08). These latter clinical event rates were related to baseline CACS and were markedly reduced in a subgroup of subjects with a baseline CACS > 400 (8.7% vs. 15.0% (42% reduction), P = 0.04).
Another study evaluated the progression of subclinical atherosclerosis in 177 asymptomatic postmenopausal women using no hormone replacement therapy (HRT), combined therapy, and estrogen alone in an observational study design. Calcium progression was 14.6 ± 21% in women taking any hormone therapy ( n = 97), whereas calcium progression rates in nonusers ( n = 80) was higher at 22.3 ± 32%. Relative to the nonuser group, HRT treatment inhibited the progression of atherosclerosis by 35% ( P = 0.01). Of the women on HRT ( n = 97) on estrogen replacement only ( n = 62), the annual increase in calcium scores was 63% lower (9 ± 22%) compared to those on estrogen plus progestin, rates similar to the nonuser group. These findings were independent of age, CV risk factors, statin use, or baseline CACS. The results of this observational CT study align with the Women Health Initiative, demonstrating that combined estrogen plus progestin imparts a significant increase in CV risk in asymptomatic women.
Based on these results, it is plausible that a baseline calcium scan assessment may hold clinical value in the prediction of future CV risk and guiding initiation of anti-lipid therapy (independent of serum lipid profile). Additional longitudinal studies addressing this issue need to be performed.
8.3.2
Coronary CT angiography
Coronary CT angiography (CCTA) is a noninvasive technique to image the coronary arteries, whereby the identification of not only coronary calcium but also coronary luminal stenosis afford valuable diagnostic and prognostic utility [ ]. Over the past decade, technological improvements in spatial resolution (225–750 μm) and temporal resolution (75–125 ms), allow visualization of the epicardial coronary arteries in a 1–5 s breath hold. Indices of diagnostic accuracy for stenosis detection exhibit a sensitivity of 95% and specificity of 83%, on a per-patient level [ ].
Despite these technological advances in CCTA, the risk of renal dysfunction (from iodinated contrast) and exposure to ionizing radiation exist. However, these radiation-related risks have been ameliorated with various technical innovations, including ECG-triggered roentgen output modulation, body-size-dependent tube voltage modification, prospective ECG-triggered scan protocols, shorter scan ranges, iterative reconstruction algorithms, and automatic exposure control [ , ]. These measures allow for routine cardiac CT at radiation doses of ~ 2–4 mSv, but may be reduced below 1 mSv in selected patients.
8.3.2.1
CCTA and cardiovascular risk
CCTA is also a robust noninvasive imaging modality that can directly visualize atherosclerotic plaque. Similar to coronary calcium, the presence of atherosclerosis on CCTA is associated with a worse clinical outcome. Although the number of > 50% stenosed vessels has the strongest predictive value, it has been demonstrated that patients with < 50% obstructive atherosclerotic plaque also have a worse outcome compared to patients with normal coronary arteries. For example, the CCTA Evaluation for Clinical Outcomes (CONFIRM), a large, prospective, open-label, international multicenter dynamic observational registry of patients who underwent CCTA [ ], demonstrated the prognostic value of CCTA findings, with a dose–response relationship of increasing extent of CAD, severity of coronary luminal stenosis, to incident mortality and MACE ( Figure 8.2 ), with prognostic value beyond traditional risk factors (TRFs). In a meta-analysis of 42 studies, the sensitivity and specificity of CCTA to identify any coronary arterial plaque was 93% and 92%, respectively [ ]. Despite the robust ability to noninvasively identify coronary atheroma, at this moment, there are no clear recommendations on the risk interpretation and subsequent management of patients with (nonobstructive) coronary plaque.
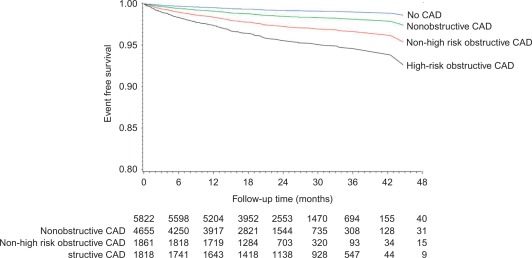
In addition to quantifying plaque burden, CCTA affords detailed imaging of the coronary arterial wall, and thus, anatomical characterization of atherosclerotic plaque. High-risk plaque features such as, low attenuation plaque, i.e., attenuation values below 30HU, positive remodeling, and the napkin-ring sign, i.e., low plaque core attenuation value with a rim of higher attenuation, have been shown to correlate with worsened prognosis [ , ] ( Figure 8.3 ). In one such study, CCTA was performed in human donor hearts to determine the histologic corollaries of advanced coronary atherosclerotic plaques. In a multivariate analysis, necrotic core area (OR = 1.9), non-core plaque area (OR = 1.6), and total vessel area (OR = 0.9) independently predicted the appearance of the napkin-ring sign (a high-risk plaque feature) on CCTA. In another study, plaque composition by CCTA was shown to enhance the predictive capacity for adverse coronary events [ ]. In a study of 368 patients, who presented with acute chest pain and had documented stenosis on CCTA, a CT-based score of morphologic coronary lesion characteristics (positive remodeling, spotty calcification, stenosis length, plaque volume) had good discriminatory value for detecting ACS during hospital admission. Additional studies have demonstrated that characterization of atherosclerotic plaque provides incremental predictive value for CV events independent of coronary stenosis and traditional risk factors alone [ ]. The ability of CCTA to delineate plaque composition has been shown to strongly correlate with intravascular ultrasound (IVUS), optical coherence tomography (OCT) and histology.
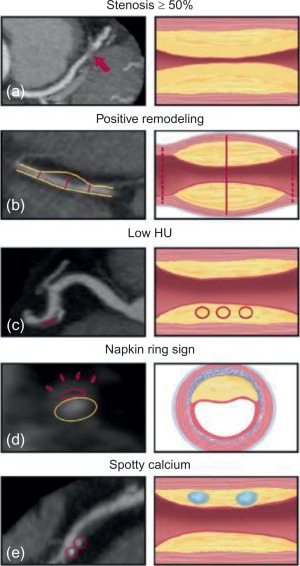
8.3.2.2
Emerging tissue and biological characterization techniques with CT
A major focus of molecular imaging technology has been on identifying high-risk atheroma. Though this approach has been borne out mostly with PET imaging (to be discussed later), there is emerging data that CT molecular imaging holds promise for high-risk plaque identification. Based on the fact that macrophages play a key role in atherogenesis and progression, in a preclinical model of atherosclerosis, the CT iodine-based contrast agent N1177, which accumulates in macrophages, was tested to determine its ability to localize to plaque in vivo and allow for it detection noninvasively with CT [ ]. This study demonstrated that N1177-enhanced CT showed increased aortic wall enhancement in atherosclerotic rabbits compared to control rabbits (10.0 ± 5.2 vs. 2.0 ± 2.1 HU, respectively; P < 0.05). In addition, the atherosclerotic rabbits underwent [ ] FDG, PET imaging, which demonstrated higher standardized uptake values (SUV) in the aortic wall of versus controls (0.61 ± 0.12 vs. 0.21 ± 0.02; P < 0.05). Altogether, the intensity of enhancement in the aortic wall measured with N1177-CT correlated with FDG uptake/ ( r = 0.61, P < 0.001) and macrophage density on immunohistology ( r = 0.63, P < 0.001).
In another study, spectral CT or multicolor CT was used in combination with gold HDL nanoparticle contrast agent (Au-HDL), for characterization of macrophage burden, calcification, and stenosis of atherosclerotic plaques [ ]. Apolipoprotein E knockout (apo E-KO) mice were used as the model for atherosclerosis, while wild-type mice served as controls. Macrophage targeting by Au-HDL was confirmed by transmission electron microscopy and confocal microscopy of aortic sections. The results showed that multicolor CT enabled differentiation of Au-HDL accumulation in the aorta. Microscopy of aortic sections revealed that Au-HDL was primarily localized in macrophages. The results of these studies show that CT, when paired with contrast agents, may yield valuable information about atherosclerotic plaque composition. Pilot studies in humans are needed to determine translatability of the findings from the above studies.
8.3.2.3
CCTA and clinical trials
CCTA plaque imaging has so far not been used extensively as a (surrogate) endpoint in large clinical trials. Reproducibility of coronary plaque quantification by CCTA between observers and particularly between examinations has been a challenge as a result of limited (spatial) resolution in relation to the small volumetric changes over time, although improvement has been made with contemporary technology. In a phase-2 multicenter study of patients with recent ACS, CCTA performed at baseline and 24 weeks demonstrated reductions in new coronary plaque formation and noncalcified plaque volumes, in those randomized to anti-inflammatory 5-lipoxygenase inhibitor therapy [ ]. In another study, serial CCTA was performed in 32 patients (26 men, ages 64.3 ± 8.5 years) to investigate the effect of statin treatment on coronary plaque morphology. Twenty-four patients received fluvastatin after the baseline CCTA, and eight subjects who refused statin treatment served as controls. After a median interval of 12 months, all vessels were examined, and demonstrated that statins decreased low attenuation plaque volume. Similar findings were seen in the New Age II Pilot Study, whereby treatment with atorvastatin 20 mg significantly reduced noncalcified plaque burden (23 ± 14% annual decrease). Other studies using CCTA to evaluate interval plaque modulation in response to statins are ongoing.
8.3.3
Magnetic resonance imaging
Magnetic resonance imaging (MRI) is a noninvasive, nonionizing imaging modality that provides multiparameter and multiplanar 3D data. The physics of MRI are extensively described in Chapter 6 . Briefly, MRI is based on the resonance of protons, which have been subjected to a magnetic field upon exposure to radiofrequency pulse. The MR signal intensity from the relaxation time of the protons is governed by the biochemical environment of protons in the respective tissue. Specific weighted spectral sequences, including T1, T2, and proton density, provide high contrast and allows for tissue characterization. These different contrasts allow for separating tissues based on their chemical and biological composition [ ]. In regard to atherosclerosis imaging, MR angiography uses time-of-flight or contrast-enhanced sequences to provide a high signal and resolution, which produces a bright lumen signal, allowing for the assessment of luminal arterial stenosis. Current MRI scanners can achieve in-plane spatial resolution of 250 × 250 μm 2 for the carotid arteries, 800 × 800 μm 2 for the aorta, and 460 × 460 μm 2 for the coronary arteries with a slice thickness of 2–5 mm [ ].
However, a significant advance of MRI rests in its ability to perform cross-sectional arterial imaging and to characterize plaque components within the vessel wall. For example, black-blood imaging, by suppressing the signal of the blood, provides a high contrast between the interface of the vessel wall and lumen, allowing for arterial wall measurements and plaque assessment. Data from multiple contrast weightings enables characterization of fibrosis, lipid cores, hemorrhage, or calcification within atherosclerotic plaque.
Beyond the anatomical information provided by morphological spectral sequences, the use of dynamic contrast-enhanced sequences allows for the assessment of functional information as it relates to plaque pathobiology. Neovascularization and macrophage accumulation are characteristic biological effects of inflammation within an atherosclerotic plaque [ , ]. MRI contrast agents migrate away from the blood pool and diffuse into plaque, thereby modifying the relaxation time. Gadolinium accumulates in interstitial tissues and enhances the signal, whereas nanoparticulate contrast agents such as ultrasmall superparamagnetic particles of iron oxide (USPIO) accumulate in macrophages and decrease the signal intensity [ ]. Blood-suppression techniques, in addition to fast scanning sequences, have promulgated the development of 3D isotropic sequences, resulting in greater signal-to-noise ratio and high spatial resolution to 0.7 mm [ ], aiding better characterization of plaque constituents.
Furthermore, increase in Tesla field strength (i.e., 1.5-T to 3-T) improves the signal-to-noise ratio [ ] for plaque morphology and composition identification, and shorter scan times. Lastly, MRI’s major advantage compared to other imaging modalities is the absence of ionizing radiation facilitating its use to serially monitor disease progression and therapeutic efficacy [ ].
8.3.3.1
MRI and cardiovascular risk
Plaque characterization by MRI has been histologically validated in humans in the carotid arteries after endarterectomy [ ]. MRI’s ability to provide quantitative measures on plaque size and composition highlights MRI’s potential to yield prognostic information about future clinical events (REFs). Studies have demonstrated that MRI is superior to biomarkers for the identification of subclinical atherosclerosis [ ]. In this regard, MRI may potentially lead to refinement of traditional CV risk stratification calculators for future MACE in asymptomatic patients [ ].
MRI-based prospective studies [ ] have shown that atherosclerotic plaque characteristics associated with future clinical events were thin fibrous caps (hazard ratio [HR] 17.0), intraplaque hemorrhage (HR 5.2), lipid/necrotic core area (HR for 10% increase 1.6), and maximum wall thickness (hazard ratio for a 1-mm increase, 1.6). More recent studies have underlined the critical importance of intraplaque hemorrhage in the prediction of downstream clinical events [ ] ( Figure 8.4 ).
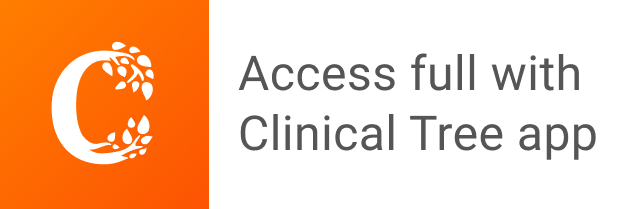